Magnets
Like electricity, magnetism is an important part of daily life. We use it to post notes on the refrigerator and to figure out which way is north. The story of magnetism wouldn't be complete, though, without including electricity. The moving electric charges give rise to magnetism, and changing magnetism gives rise to electricity. How would a family stay organized without refrigerator magnets? How would the doorbell ring if it couldn't use a magnet to strike its bells? How would a scout navigate in the woods without a compass? How would you get cash or charge purchases without magnetic strips on plastic cards? We're so used to having magnets around that we take them for granted. Along with being useful, household magnets also let us experiment with another of the basic forces in nature. Although we'll see that magnetism is so intimately related to electricity that the two are ultimately a single unified whole, we'll find it helpful to begin our study of magnetism by treating it as a separate phenomenon and bring electricity into the picture gradually.
Button-Shaped Refrigerator Magnets
Refrigerator magnets come in all shapes and sizes. As you bring two button magnets together, they begin exerting forces on one another. Magnetism is a phenomenon that closely resembles electricity. Just as there are two types of electric charges that exert electrostatic forces on one another, so there are two types of magnetic poles that exert magnetostatic forces on one another. The word pole serves to distinguish magnetism from electricity; poles are magnetic, while charges are electric.
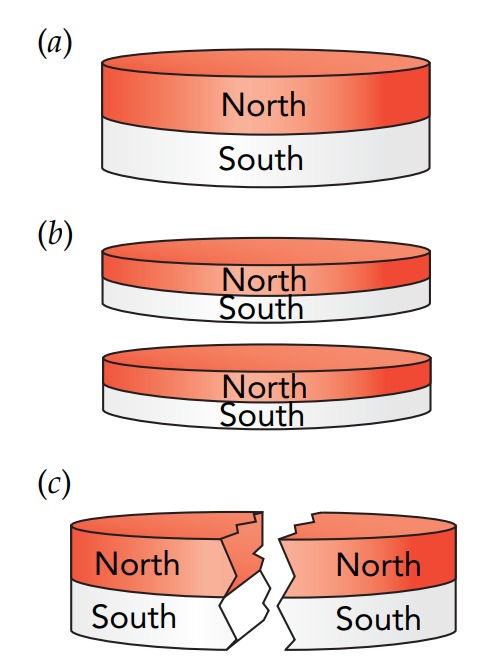
The two types of poles are called north and south, and in keeping with this geographical naming, they're exact opposites of one another. Both types of poles carry just one physical quantity: magnetic pole. North poles carry positive amounts of magnetic pole, while south poles carry negative amounts. It should come as no surprise that like poles repel each other, while opposite poles attract. Furthermore, the magnetostatic forces between two poles grow weaker as they move apart and are inversely proportional to the square of the distance between them. So far, the similarities between electricity and magnetism are striking.
The crucial difference between electricity and magnetism is the absence of magnetic monopoles. While subatomic particles that carry pure positive or negative electric charges are common, particles that carry pure north or south magnetic poles have never been found. Called magnetic monopoles, such pure magnetic particles may not even exist in our universe. That cosmic omission explains why there are no magnetic sparks: without monopoles, there is no magnetic equivalent of an electric charge that can leap from one place to another as a magnetic current, let alone a magnetic spark.
Although isolated magnetic poles aren't available in nature, pairs of magnetic poles are. These pairs consist of equal north and south poles, spatially separated from one another in an arrangement called a magnetic dipole. Since the two opposite poles have equal magnitudes, they sum to zero and the magnetic dipole has zero net magnetic pole. A simple button magnet has both a north pole and a south pole, usually on opposite faces of the button. Amazingly enough, even slicing that button magnet in half won't yield separated north and south poles. Instead, new poles will appear at the cut edges and each piece of the original magnet will end up with zero net pole! Breaking the button magnet in half will also produce pieces with zero net pole.
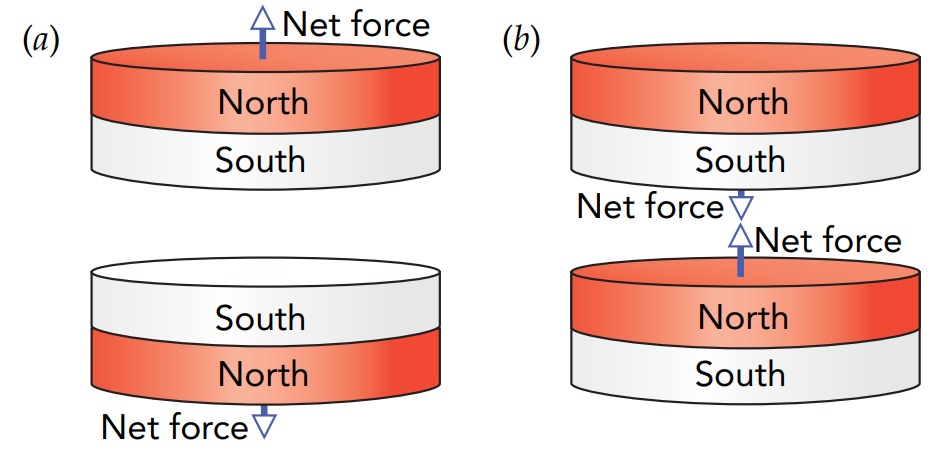
With two poles on each magnet, we have to consider four interactions: two repulsive interactions between like poles (north-north and south-south) and two attractive interactions between opposite poles (north-south and south-north). Although it might seem that all these forces should cancel, the distances separating the various poles and therefore the forces between them depend on the magnets' orientations. Since the closest poles experience the strongest forces, they dominate. If you turn two like poles toward one another, the two magnets will push apart. If you turn their opposite poles toward one another, they'll pull together. If you tip them at an angle, they'll experience torques that tend to twist opposite poles together and like poles apart. The SI unit of magnetic pole is the ampere-meter (abbreviated A·m). That astonishing choice, an electric unit appearing in a magnetic unit, foreshadows the profound connections between electricity and magnetism.
The forces between magnetic poles are proportional to the amount of each pole and inversely proportional to the square of their separation. The exact relationship can be written as a word equation:
\[ force = \frac{permeability~ of~ free ~space · pole_1 · pole_2}{4\pi · (distance ~between~ poles)^2} \]
in symbols:
\[F = \frac{\mu_0}{4\pi}\frac{p_1 · p_2}{r^2} \]
and in everyday language:
Don't hold two strong magnetic poles near one another unless you're prepared to be pushed around hard as they attract or repel each other.
The permeability of free space is \(4\pi × 10^{−7} N/A^{2}\).
The Refrigerator: Iron and Steel
Two button magnets can push or pull on one another. The easiest way to observe magnetic forces in that case is to hold that single magnet near your refrigerator or another piece of iron or steel. The magnet is attracted to the refrigerator. However, if you flip the button magnet over, thinking that it will now be repelled by the refrigerator, you'll be disappointed. Although the refrigerator is clearly magnetic, its magnetism somehow responds to the button magnet so that the two always attract.
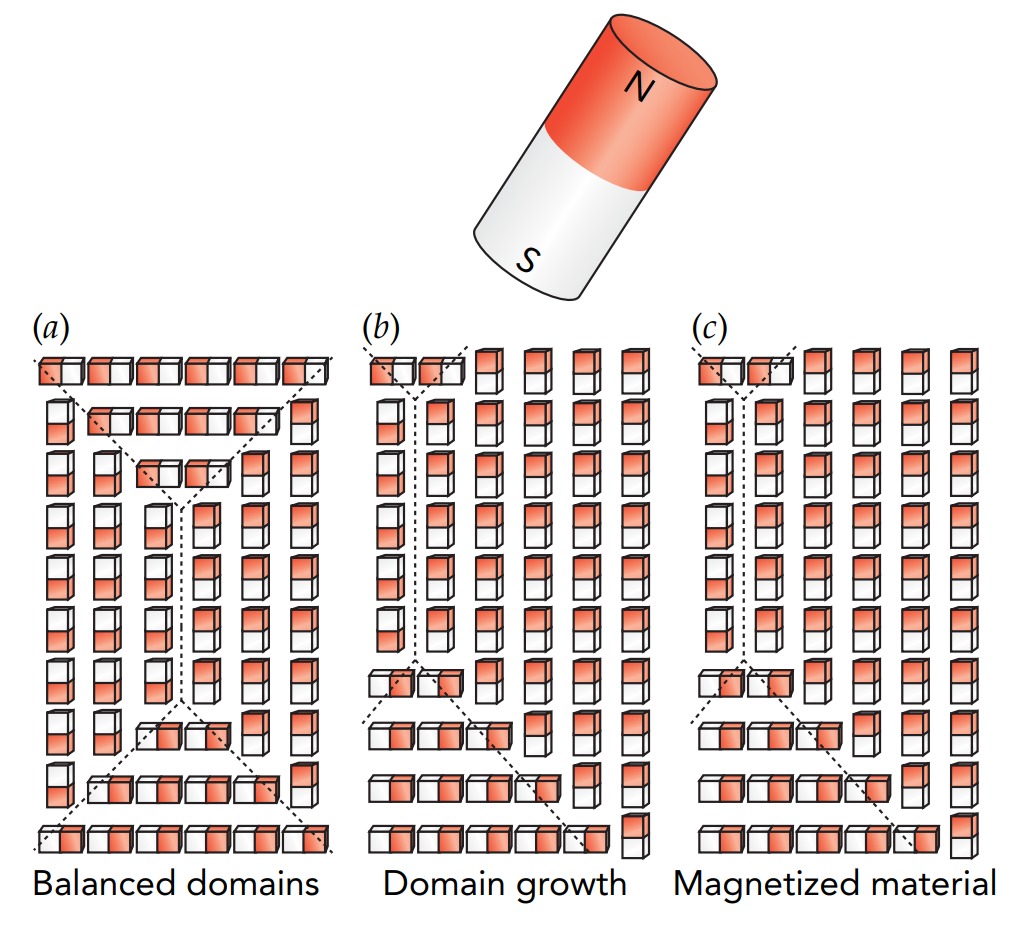
Its steel in the refrigerator which is composed of countless microscopic magnets, each with a matched north pole and south pole. Normally those individual magnetic dipoles are oriented semirandomly, so the refrigerator exhibits no overall magnetism. However, as you bring one pole of a button magnet near the refrigerator, its tiny magnets evolve in size, shape, and orientation. Overall, opposite poles shift closer to the button magnet's pole and like poles shift farther from the button magnet's pole. The steel develops a magnetic polarization and consequently attracts the pole of the button magnet.
This polarization remains strong only as long as the button magnet's pole is nearby. When you remove the button magnet, most of the tiny magnets in the steel resume their semirandom orientations and the steel's magnetic polarization shrinks or disappears. When you then bring the button magnet's other pole close to the refrigerator, its steel develops the opposite magnetic polarization and again attracts the button magnet's pole. No matter which pole or assortment of poles you bring near the refrigerator, its steel will polarize in just the right way to attract those poles.
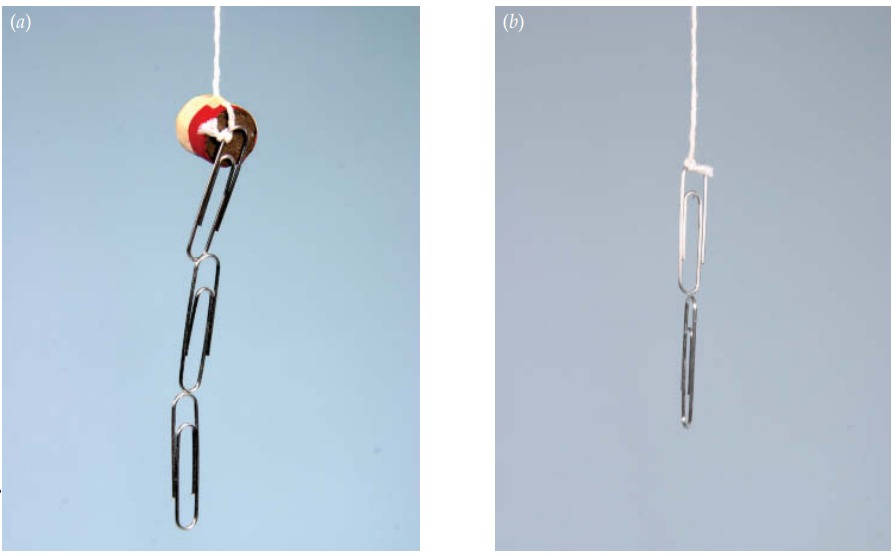
If you try this trick with a plastic or aluminum surface, the button magnet won't stick. What's special about steel that allows it to develop such a strong magnetic polarization? The answer is that ordinary steel, like its constituent iron, is a ferromagnetic material—it is actively and unavoidably magnetic on the size scale of atoms. To understand ferromagnetism, we must start by looking at atoms and the subatomic particles from which they're constructed: electrons, protons, and neutrons. For complicated reasons, all those subatomic particles have magnetic dipoles, particularly the electrons, and the atoms they form often display this magnetism. Despite a tendency for the subatomic particles to pair up with opposite orientations so that their magnetic dipoles cancel one another, most isolated atoms have significant magnetic dipoles.
Although most atoms are intrinsically magnetic, most materials are not. That's because another round of pairing and canceling occurs when atoms assemble into materials. This second round of cancellation is usually so effective that it completely eliminates magnetism at the atomic scale. Materials such as glass, plastic, skin, copper, and aluminum retain no atomic-scale magnetism at all, and your button magnet won't stick to them. Even most stainless steels are nonmagnetic.
However, there are a few materials that avoid this total cancellation and thus manage to remain magnetic at the atomic scale. The most important of these are the ferromagnets, a class of magnetic materials that includes ordinary steel and iron. If you examine a small region of ferromagnetic steel, you'll find that it is composed of many microscopic regions, or magnetic domains, that are naturally magnetic and cannot be demagnetized. Within a single domain, all the atomic-scale magnetic dipoles are aligned and together they give the overall domain a substantial net magnetic dipole.
While common steel always has these magnetic domains, magnetic interactions orient nearby domains so that their magnetic dipoles oppose one another and cancel. The microscopic magnets balance one another so well that the steel appears nonmagnetic. That's too bad; the appliance showroom would be a much more exciting place to visit if the cancellation weren't so good.
However, when you bring a strong magnetic pole near steel, the individual domains grow or shrink, depending on which way they're oriented magnetically. The steel undergoes magnetization and becomes magnetized. The atoms themselves don't move during this process; the change is purely a reorientation of the atomic-scale magnetic dipoles. Domains that attract your button magnet's pole grow while those that repel it shrink, and the button magnet sticks to the refrigerator.
Plastic Sheet Magnets and Credit Cards
When you remove your button magnet from the refrigerator, the steel returns to its original nonmagnetic state—it undergoes demagnetization and becomes demagnetized. Well, almost demagnetized. The demagnetization process isn't perfect because some of the domains get stuck. Although magnetic forces within the steel favor a complete return to apparent nonmagnetism, chemical forces can make it hard for the domains to grow or shrink. Adjacent domains are separated by domain walls, boundary surfaces between one direction of magnetic orientation and another. These domain walls must move if the domains are to change size. However, flaws and impurities in the steel can interact with a domain wall and keep it from moving. When that happens, the steel fails to demagnetize itself completely. To remove the last bit of residual magnetism from steel, you must help the domain walls move, typically with heat or mechanical shock.
A soft magnetic material is one that demagnetizes itself easily when all nearby poles are removed. Chemically pure iron, which has few flaws or impurities, is a soft magnetic material—easy to magnetize and easy to demagnetize. A hard magnetic material is one that does not demagnetize itself easily and that tends to retain whatever domain structure is imposed on it by its most recent exposure to strong nearby poles. Your button magnet is made from a hard magnetic material!
Like steel, the material in your button magnet is ferromagnetic (or closely related to ferromagnetic). Unlike steel, however, your button magnet's domains do not shrink or grow easily. During its manufacture, the button magnet was magnetized by exposing it to such strong magnetic influences that its domains rearranged to give it permanent magnetic poles. It now has a north pole on one face and a south pole on the other. Unless you expose the button to extremely strong magnetic influences or heat it or pound it, it will retain its present magnetization almost indefinitely. In that respect, the button is a permanent magnet.
Not all permanent magnets are as simple as button magnets. Depending on how they were magnetized, they can have their north and south poles located in unexpected places and even have more than one pair of poles. Plastic sheet magnets are a good example of multiple-pole magnets: each has a repeating pattern of alternate poles along its length. The exact patterns vary, but most have poles that form alternating parallel stripes. You can find these stripes by letting them polarize and attract iron powder or by sliding two identical sheet magnets across one another. The sheets will attract and bind together most strongly when opposite poles are aligned across from each other. They'll repel when you shift one of the magnets so that like poles are aligned.
A hard magnetic material's ability to “remember” its magnetization can be useful for saving information. Once magnetized in a particular manner so as to represent a piece of information, the material will retain its magnetization and the associated information until something magnetizes it differently. Information retention in hard magnetic materials forms the basis for most magnetic recording and storage, including the magnetic strips on credit cards, magnetic tapes, computer disks, and magnetic random access memory (MRAM)
Compasses
If you've spent time hiking, you may well own a magnetic compass. Like a button magnet, the needle of that compass is a simple permanent magnet with one north magnetic pole and one south magnetic pole. This needle aids navigation because Earth itself has a magnetic dipole and that dipole affects the orientation of the needle—the needle's north magnetic pole tends to point northward.
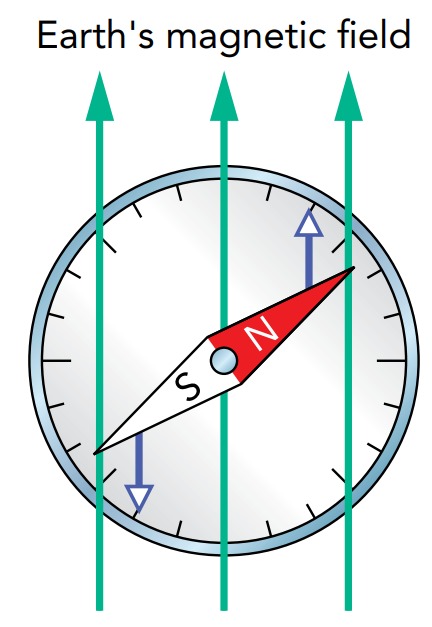
Already, we can guess what must be located near Earth's north geographic pole—a south magnetic pole. Attraction from that south magnetic pole is what draws the compass's north magnetic pole toward the north. However, the full story is more complicated. To begin with, Earth's magnetic poles are actually located far beneath its surface and aren't perfectly aligned with the geographic poles. To make matters worse, magnetically active materials in everything from distant mountains to nearby buildings assert their own magnetic influences on the compass needle. Overall, the compass needle is responding to the influences of countless magnetic poles, both near and far. Given how difficult it would be to sum up all those separate influences, it is easier to view the compass needle as interacting with something local—a magnetic field, an attribute of space that exerts a magnetostatic force on a pole. According to this new perspective, the compass needle responds to the local magnetic field, a field that's created by all the surrounding magnetic poles.
As with an electric field, the magnetic field here appears to be acting merely as an intermediary; various poles produce the magnetic field, and this magnetic field affects our compass needle. As we'll see, however, a magnetic field is more than just an intermediary or a fiction. It is quite real and can exist in space, independent of the poles that produce it. Just as electric fields can be created by things other than charge, so magnetic fields can be created by things other than pole. The magnetic field at a given location measures the magnetostatic force that a unit of pure north pole would experience if it were placed at that point. More specifically, the magnetostatic force is equal to the pole times the magnetic field at the pole's position. We can write this relationship as a word equation:
\[magnetostatic~ force = pole · magnetic~ field\]
in symbols:
\[F = pB\]
and in everyday language:
If you place a strong magnet in a big magnetic field, expect to be pushed around,
where the magnetostatic force is in the direction of the magnetic field. Note that a negative amount of pole (a south pole) experiences a force opposite the magnetic field. The SI unit of magnetic field is the newton per ampere-meter, also called the tesla (abbreviated T).
Earth's magnetic field is relatively weak, about 0.00005 T in a roughly northward direction. Earth's magnetic poles are not particularly well aligned with its geographical poles and have actually moved about 1100 km over the past century. Earth's south magnetic pole is presently drifting northwest across the Canadian arctic at about 40 kilometers per year. Moreover, Earth's magnetic poles have been trading places about once every 700,000 years for the past 330 million years. The most recent reversal was about 780,000 years ago.
Earth's field pushes the compass needle's north pole northward and south pole southward. Unless the compass needle is perfectly aligned with that field, it experiences a torque and undergoes angular acceleration. Since its mount allows the needle to rotate only horizontally and it experiences mild friction as it does, the needle soon settles down with its north pole pointing roughly northward. If its mount allowed it to rotate vertically as well as horizontally, the needle's north pole would dip downward in the northern hemisphere and upward in the southern hemisphere. In general, the needle minimizes its magnetostatic potential energy by pointing along the direction of the local magnetic field and is thus in a stable equilibrium when orientated that way. After a few swings back and forth, your compass needle points along the local magnetic field, which, we hope, points northward.
Because Earth's magnetic field is so uniform in the vicinity of your compass, its northward push on the needle's north pole exactly balances its southward push on the needle's south pole and the needle experiences zero net force. However, if you bring your compass near a button magnet, the local magnetic field will not be uniform and the needle may experience a net force. The magnetic field gets stronger near one of the button's poles and the compass needle will experience a net force toward or away from that pole, depending on which way it's orientated.
When the needle is aligned with a nonuniform field—its north pole pointing in the same direction as the local field—the forces on its two opposite poles won't balance and it will experience a net force in the direction of increasing field. If it is aligned against the field, it will experience a net force in the direction of decreasing field. In practice, as you bring the compass near your button magnet, its needle will first pivot into alignment with the local field and then find itself pulled toward increasing field, toward the nearest pole of the button magnet. The same thing happens when you bring two button magnets together; each pivots into alignment with the other's magnetic field, and the two then leap at each other. Watch out for your fingers!
A piece of steel exhibits similar behavior when you hold it near a button magnet: it becomes magnetized along the direction of the local magnetic field and then finds itself pulled toward increasing field, toward the button magnet's nearest pole. That's how the button magnet holds your notes to the refrigerator!
Iron Filings and Magnetic Flux Lines
Magnetic fields seem abstract; it would be helpful if you could see them. Remarkably enough, you can—just sprinkle iron filings into the field! Although you'll need to support their weight with paper or a liquid, an interesting pattern will form. Like tiny compass needles, the iron particles magnetize along the local magnetic field and then stick together, north pole to south pole, in long strands that delineate the magnetic field! These strands map the magnetic field in an interesting way. First, at each point on a strand, the strand points along the local magnetic field. Second, the strands are most tightly packed where the local magnetic field is strongest. In other words, the strands follow along the local magnetic field direction and have a density proportional to that local field. The lines highlighted by these strands are so useful that they have their own name, magnetic flux lines.
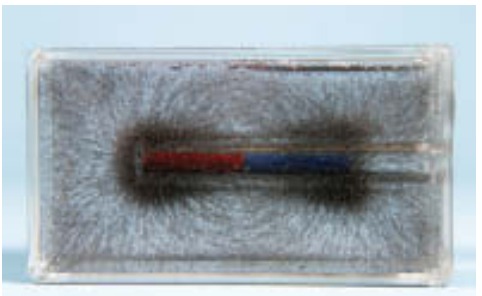
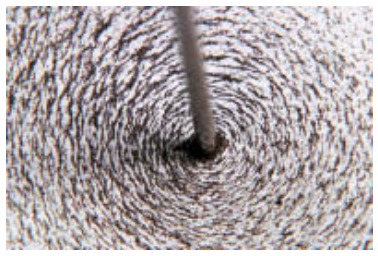
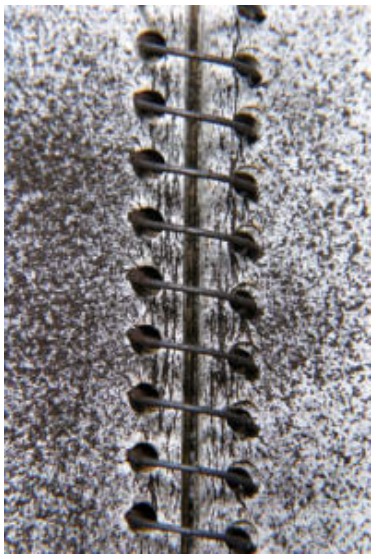
Flux lines are often helpful when exploring a magnetic field. If you're studying the magnetic field in a large area, you probably don't want to use iron filings. Instead, you can hold a compass in your hand and walk in the direction its needle is pointing—the direction of the magnetic field. The path you'll follow in this compass-guided walk is a magnetic flux line. If you repeat this trip from many different starting points, you'll explore the whole magnetic field, flux line by flux line. Since a magnetic field tends to point away from north poles and toward south poles, these tours will typically take you from north poles to south poles. In fact, for our permanent magnets, every magnetic flux line begins at a north pole and ends at a south pole.
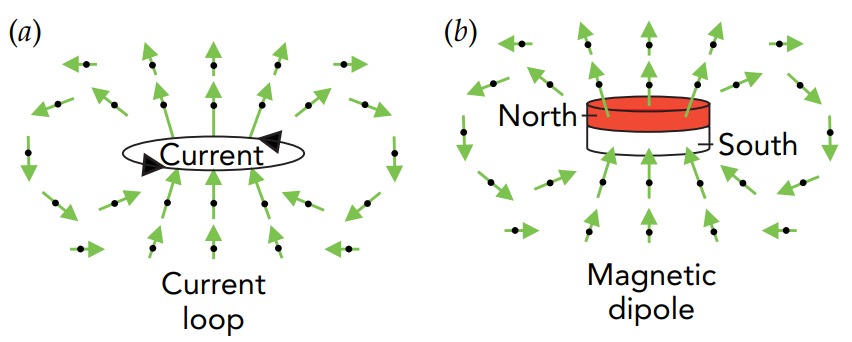
That last observation about flux lines is quite general: they never start at or end on anything other than a magnetic pole. While flux lines emerge in all directions from a north pole and converge from all directions on a south pole, that's it; flux lines never begin or end in empty space. If you're following a magnetic flux line with your compass, you will either reach a south pole or walk forever!
Electric Doorbells and Electromagnets
A classic electric doorbell uses a magnet and a spring to drive a piece of iron into two chimes, “ding-dong.” When you press the doorbell button, you close an electric circuit and the resulting electric current pushes the iron magnetically into the first chime, “ding.” When you release the button, you open the circuit, stopping the current and its magnetism so that the spring can push the iron back into the second chime, “dong.”
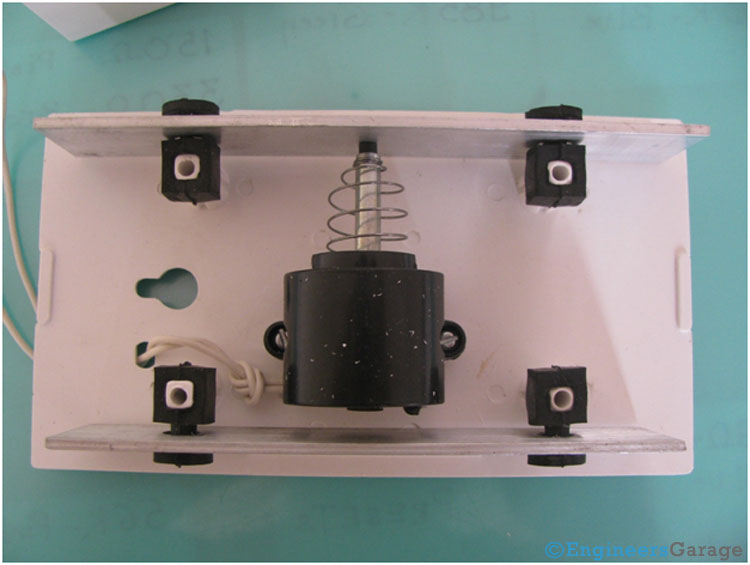
Imagine the surprise of Danish physicist Hans Christian Oersted (1777–1851) when he observed in 1820 that current in a wire caused a nearby compass needle to rotate. Until that moment, electricity and magnetism had appeared to be independent phenomena. Inspired by Oersted's experiment, French physicist André-Marie Ampère 3 undertook a 7-year study of the relationships between electricity and magnetism, and started the revolution that eventually unified them within a single overarching conceptual framework.
When we use iron powder to disclose the magnetic flux lines surrounding a long, straight, current-carrying wire, we too are in for a sur. Those flux lines circle the wire like concentric rings, growing more widely separated as the distance from the wire increases. The wire is an electromagnet, a device that becomes magnetic when it carries an electric current. However, because an electromagnet has no true magnetic poles, the magnetic flux lines can't stretch from north pole to south pole. Instead, each flux line of an electromagnet is a closed loop. If you took a compass-guided walk along one of these flux lines, you'd retrace your steps over and over. Since the flux lines are packed tightest near the surface of the current-carrying wire, that's where the magnetic field is strongest. Recalling that a piece of iron is pulled toward increasing magnetic field, we see that the wire will attract iron to it whenever it's carrying a current.
The magnetic field around a current-carrying wire is fairly weak, however, and a practical doorbell winds that wire into a coil to concentrate and strengthen its field. Although the magnetic field around a current-carrying coil is complicated, we can use iron powder to make it visible. Remarkably enough, the flux lines outside the coil resemble those outside a button magnet of similar dimensions. It's as though the coil has a north pole at one end and a south pole at the other! Because there are no true poles present, however, the flux lines don't end anywhere. Instead, they continue right through the middle of the coil and form complete loops.
When current flows through the coil, nearby iron finds itself magnetized along the local magnetic field and then pulled toward increasing field—toward the tightly packed flux lines at the coil's end. But why stop there? Since the flux lines continue right into the coil and grow even more tightly packed inside, the iron will be pulled inward toward the very center of the coil!
That's how the doorbell works. When you press the doorbell button, current flows through a coil of wire and the resulting magnetic field yanks an iron rod into the center of that coil. About the time the rod reaches the center, part of it hits the first chime. When you then open the switch, stopping the current and its magnetism, a spring pushes the iron rod back out of the coil and it hits the second chime. These two chimes make the familiar dingdong! While current is flowing through the coil and the iron rod is inside it, the two objects act as a single powerful electromagnet. The magnetic field surrounding the pair is the sum of the coil's modest magnetic field and the magnetized iron's much stronger field. In effect, the current in the coil magnetizes the iron and the iron then creates most of the surrounding magnetic field. Practical electromagnets, which control switches and valves in your furnace or air conditioner and can lift cars at the scrap yard, generally use iron or related materials to dramatically enhance the magnetic field produced by a current in a coil of wire.
Electric Power Distribution
Electricity is a particularly useful and convenient form of ordered energy. Because it's delivered to our homes and offices as a utility, we barely think about it, except to pay the bills. The wires that bring it to us never plug up or need cleaning and work continuously except when there's a power failure, blown fuse, or tripped circuit breaker.