We can’t see all the motion that takes place around us. Some of it is hidden deep inside each object, where thermal energy keeps the individual atoms and molecules jiggling back and forth in an endless flurry of activity. We’re usually aware of this thermal energy only because it determines an object’s temperature; the more thermal energy an object contains, the higher its temperature and the hotter it feels.
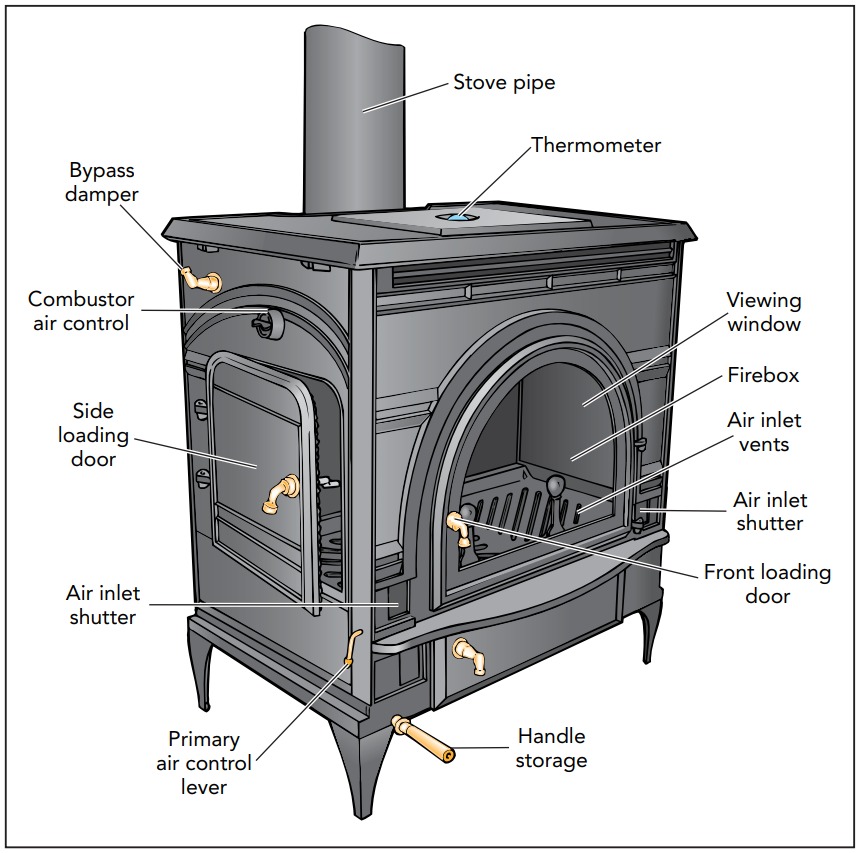
Nevertheless, thermal energy plays an important role in everyday life. In addition to moving from one place to another, thermal energy can transform a substance from a solid to a liquid to a gas. What you’re feeling when you touch a hot object is actually its thermal energy flowing into your comparatively colder hand and raising the temperature of your skin. When thermal energy is flowing in this manner, from a hotter object to a colder one, we call it heat.
A Burning Log: Thermal Energy
A woodstove produces thermal energy and distributes it as heat to the surrounding room. When you burn a log in the fireplace or woodstove, you’re turning the log’s ordered chemical potential energy into disordered thermal energy—energy contained in the kinetic and potential energies of individual atoms and molecules. The presence of thermal energy in the log, the woodstove, or the room air is what gives it a temperature; the more thermal energy it has, the higher its temperature.
In the hot burning log, thermal energy is mostly in the wood’s atoms and molecules, which jitter back and forth rapidly relative to one another. When each of these particles moves, it has kinetic energy. When it pushes or pulls on its neighbors, it has potential energy.
In the air near the burning log, thermal energy is again mostly in the atoms and molecules. However, since those particles are essentially free and independent, most of this thermal energy is kinetic energy. The air particles store potential energy only during the brief moments when they collide with one another.
In the metal poker that you use to stir the fire, thermal energy is not only in the atoms and molecules but also in the mobile electrons that move about the metal and allow it to conduct electricity. Thermal energy is the portion of internal energy that’s associated with temperature. Internal energy excludes any energy due to external forces or overall motion, so lifting the poker to increase its gravitational potential energy or waving it to increase its kinetic energy does not affect either its internal energy or its thermal energy.
Forces between Atoms: Chemical Bonds
To understand how a burning log produces thermal energy, let’s take a look at bonds between atoms and the chemical potential energy that’s stored in those bonds. Since both result from the forces between atoms. As you bring two atoms close together, they exert attractive forces on one another. These chemical forces are primarily electromagnetic in origin and grow stronger as the atoms approach. The attraction diminishes, however, when the atoms start to touch and is eventually replaced by repulsion when the atoms are too close. The separation between atoms at which the attraction ends and the repulsion begins is their equilibrium separation—that is, the separation at which the atoms exert no forces on one another. Since atoms are tiny, this equilibrium separation is also tiny, typically only about a ten-billionth of a meter.
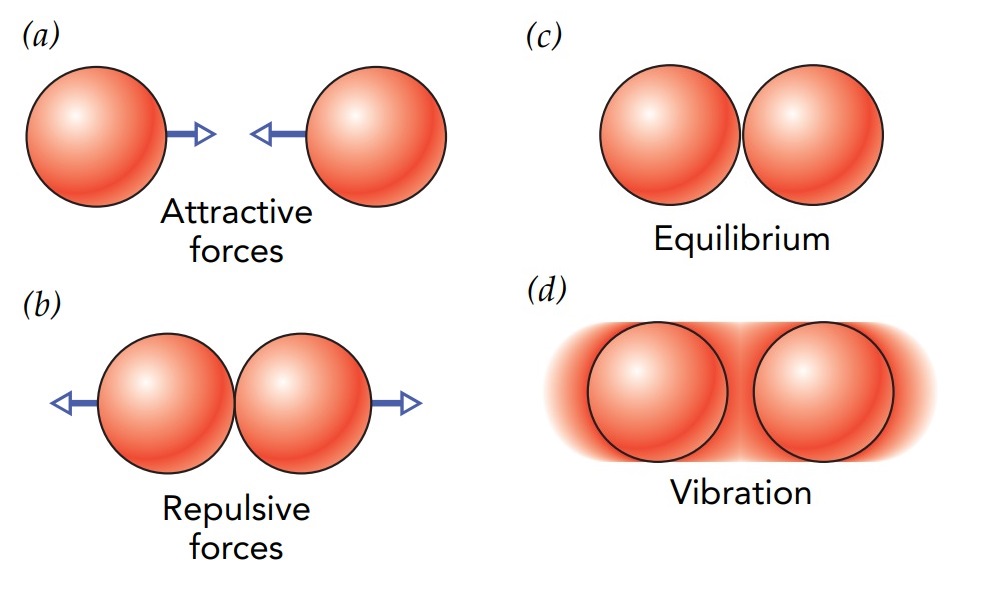
Imagine holding two atoms in tweezers and slowly bringing them together. They pull toward one another as they approach, doing work on you and increasing your energy. Since energy is conserved, their energy must be decreasing. They’re giving up chemical potential energy, energy stored in the chemical forces between atoms.
Once the atoms reach their equilibrium separation, you can let go of them and they won’t come apart. Since they’ve given up some of their chemical potential energy, they can’t separate unless that energy is returned to them; it takes work to drag them apart. Without that energy, the atoms are held together by an energy debt known as a *chemical bond. The bound atoms have become a molecule. The strength of their bond is equal to the amount of work the atoms did when they drew together or, equivalently, the work required to separate them. Bond strengths range from extremely strong in the case of two nitrogen atoms to extremely weak in the case of two neon atoms.
If they have a little extra energy, bound atoms can vibrate back and forth about their equilibrium separation. Whenever the atoms are moving quickly toward or away from one another, most of their energy is kinetic. Whenever the atoms are traveling slowly while turning around, most of their energy is chemical potential. Overall, the molecule’s total energy remains constant, and it vibrates back and forth until it transfers its extra energy elsewhere.
Many molecules have more than two atoms. In a large molecule, many pairs of atoms have chemical bonds and equilibrium separations. If you give this molecule excess energy, it will vibrate in a complicated manner as the energy moves among the various atoms and chemical bonds. The atoms in the molecule will continue to jiggle until something removes the excess energy from the molecule.
Like all liquids and solids, our burning log is just a huge assembly of atoms and molecules, held together by chemical bonds of various strengths. These particles push and pull on one another as they vibrate about their equilibrium separations. Their motion is thermal motion, and the energy involved in this disorderly jiggling is thermal energy. Because thermal energy is fragmented among the atoms and exchanged between them unpredictably, it can’t be used directly to do work.
Heat and Temperature
Everything contains thermal energy, from the hot burning log to the cold metal poker you use to stir the fire. When they touch, the poker and the log begin to exchange thermal energy. In effect, the two objects become one larger object, and thermal energy that has been moving about randomly among the particles in each individual object begins to move about randomly among the particles of both objects. Well, not quite randomly. Although tiny portions of thermal energy move in both directions between the two objects, those exchanges don’t cancel. Overall, there is a net flow of thermal energy from the hot log to the cold poker. If no thermal energy flows when two objects touch, then those objects are in thermal equilibrium and their temperatures are equal. But if thermal energy flows from the first object to the second, then the first object is hotter than the second.
A temperature scale classifies objects according to which way thermal energy will flow between any pair. An object with a hotter temperature will always transfer thermal energy to an object with a colder temperature, and two objects with the same temperature will always be in thermal equilibrium. Thus the hot burning log will transfer thermal energy to the cold poker. We say that the burning log is hot because it tends to transfer thermal energy to most objects, while the poker is cold because most objects tend to transfer thermal energy to it.
Energy that flows from one object to another because of a difference in their temperatures is called heat. Heat is thermal energy on the move. Strictly speaking, the burning log doesn’t contain heat; it contains thermal energy. However, when that log transfers energy to the cold poker because of their temperature difference, it’s heat that flows from the log to the poker.
Standard temperature scales are based on an object’s average thermal kinetic energy per atom. The more kinetic energy each atom has, on average, the more vigorous the object’s thermal motion and the more thermal energy it transfers to the atoms in a second object by way of microscopic portions of work. Microscopic work is what actually passes heat between objects. Since an object with more average thermal kinetic energy per atom will pass heat to an object with less, it makes sense to assign temperatures according to average thermal kinetic energies per atom. The Celsius, Fahrenheit, and Kelvin are the standard scales all measure temperature in this manner. In each scale, a 1-degree, or unit, increase in temperature reflects a specific increase in average thermal kinetic energy per atom. The relationship between average thermal kinetic energy per atom and assigned temperature is based on several standard conditions: absolute zero, water’s freezing temperature, and water’s boiling temperature. Once specific temperatures have been assigned to two of these standard conditions, the whole temperature scale is fixed. For example, the Celsius scale is built around 0 °C being water’s freezing temperature and 100 °C being water’s boiling temperature.
Open Fires and Woodstoves
Suppose you needed an easy way to heat your room. The oldest and simplest method would be to start a campfire in the middle of the floor. The burning wood would produce thermal energy, which would flow as heat into the colder room. This thermal energy is released by a chemical reaction between molecules in the wood and oxygen in the air.
Atoms do work as they join together in a chemical bond and that the amount of work done depends on which atoms are being joined. For example, while carbon and hydrogen atoms can bond with one another to form hydrocarbon molecules, these atoms form much stronger bonds with oxygen atoms. Thus, although it may take work to disassemble a hydrocarbon molecule, the work done by its hydrogen and carbon atoms as they bind to oxygen atoms more than makes up for that investment. As a hydrocarbon molecule burns in oxygen, new, more tightly bound molecules are formed and chemical potential energy is released as thermal energy. The reaction products produced by burning hydrocarbons in air are primarily water and carbon dioxide.
Wood is composed mostly of cellulose, a long carbohydrate molecule. Carbohydrates contain carbon, hydrogen, and oxygen atoms. Despite the presence of a few oxygen atoms, carbohydrates still burn nicely to form water and carbon dioxide. When you light the wood with a match, you’re supplying the energy needed to break the old chemical bonds so that the new bonds can form. This starting energy is called activation energy, the energy needed to initiate the chemical reaction. Heat from the match flame gives the wood enough thermal energy to break chemical bonds between various atoms and start the reaction.
Unfortunately, wood isn’t pure cellulose. It also contains many complex resins that don’t burn well and that create smoke. If you plan to breathe the air in which you burn fuel, wood is an awful choice. You’d be better off with kerosene or natural gas, both of which are nearly pure hydrocarbons and burn cleanly. Actually, wood can be converted to a cleaner fuel by baking it in an airless oven to remove all its volatile resins. This process converts the wood into charcoal, which burns to form nearly pure carbon dioxide, water vapor, and ash.
However, even with clean-burning fuels, the direct fire-in-the-room heating concept has its disadvantages—it consumes the room’s oxygen and presents a safety hazard. Nonetheless, fires have heated dwellings for thousands of years. While fireplaces that burn wood or peat have chimneys that carry away their noxious fumes, the rising smoke also takes with it much of the fire’s thermal energy and some of the room’s air. That’s why a room that’s heated by a fireplace often feels drafty away from the fireplace itself—cold outside air is seeping in through cracks to replace air drawn up the chimney. Even when clean-burning fuels are used without a chimney, there are no simple solutions to the oxygen -loss or safety problems.
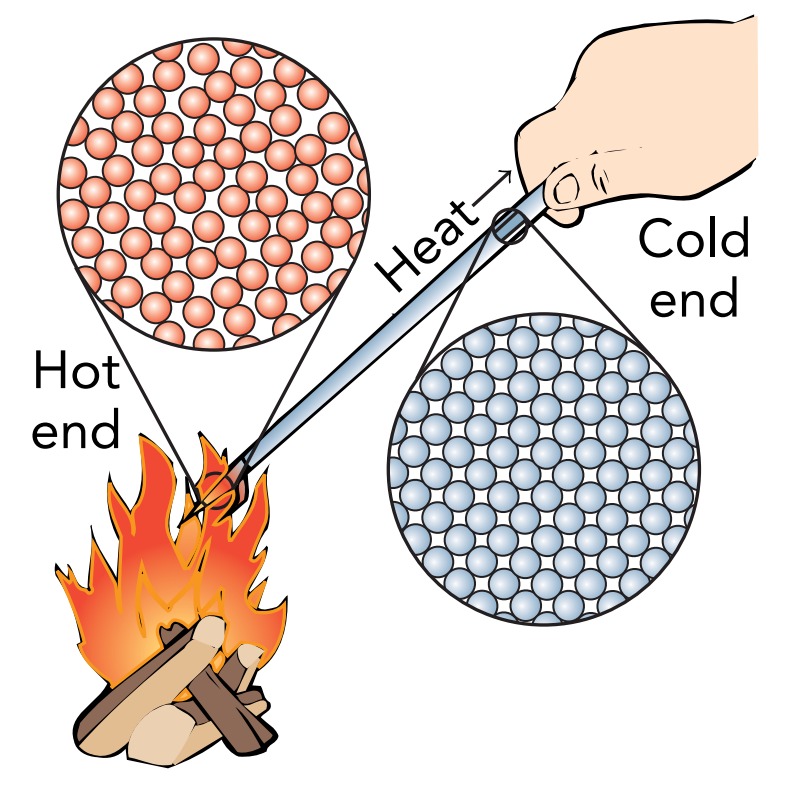
Like a fireplace, a woodstove sends fumes from its burning wood up a chimney. But before its thermal energy can follow the fumes outside, a well-designed woodstove transfers most of that energy into the room. A woodstove is an example of a heat exchanger, a device that transfers heat without transferring the hot molecules themselves. Its smoke never enters the room, but heat from that smoke does. Most combustion furnaces, such as those fueled by natural gas, propane, oil, and coal, also employ heat exchangers. The burning coals and hot gases inside the woodstove contain a great deal of thermal energy and are much hotter than the room air. Because of this temperature difference, heat tends to flow from the fire to the room. What is not so clear yet is how that heat is transferred.
There are three principal mechanisms by which heat moves from the fire to the room: conduction, convection, and radiation. The woodstove makes wonderful use of all three so that most of the thermal energy released by the burning wood is transferred to the room.
Heat Moving through Metal: Conduction
Conduction occurs when heat flows through a stationary material. The heat moves from a hot region to a cold region, but the atoms and molecules don’t. Some of this heat is conducted by interactions between adjacent atoms. The vibrating atoms frequently push on one another, doing microscopic work in the process and exchanging miniscule amounts of thermal kinetic energy. In this fashion, thermal energy flows randomly from atom to neighboring atom.
However, when the poker’s tip is hotter than its handle, the flow is no longer completely random. The atoms at the hot tip have more thermal kinetic energy to exchange with their neighbors than atoms at the cold handle. The exchanges statistically favor the flow of thermal energy away from the hot tip and toward the cold handle. This flow of thermal energy from hot to cold through the poker is conduction.
This atom-by-atom bucket brigade isn’t the only way in which materials conduct heat. In a metal, the primary carriers of heat are actually mobile electrons, the tiny negatively charged particles that make up the outer portions of atoms. When atoms join together to form a metal, some of the electrons stop belonging to particular atoms and travel almost freely throughout the metal.
Mobile electrons participate in the bucket brigade of heat conduction because they, too, can push on vibrating atoms and exchange thermal kinetic energy with them. However, while atoms can pass thermal energy only from one neighbor to the next, mobile electrons can travel great distances between exchange partners and can move thermal energy quickly from one place to another.
The ease with which electrons move heat about a metal explains why metals generally have higher thermal conductivities than nonmetals. Thermal conductivity is the measure of how rapidly heat flows through a material when it’s exposed to a difference in temperatures. The best conductors of electricity—copper, silver, aluminum, and gold—are also the best conductors of heat. Poor conductors of electricity—stainless steel and insulators such as plastic and glass—are also poor conductors of heat. There are a few exceptions to this rule. Diamonds, for example, are terrible conductors of electricity but wonderful conductors of heat.
Conduction is what moves thermal energy from the woodstove’s inside to its outside. No atoms move through the metal walls of the stove, just heat. So conduction serves as a filter, separating desirable thermal energy from the unwanted smoke and noxious gases that then go up the chimney. Thus conduction makes the outside surface of the woodstove hot, allowing heat to flow from it to the colder room.
Heat Moving with Air: Convection
Convection occurs when a moving fluid transports heat from a hotter object to a colder object. The heat moves as thermal energy in the fluid so that the two travel together. The fluid usually follows a circular path between the two objects, picking up heat from the hotter object, giving it to the colder object, and then returning to the hotter object to begin the cycle again.
This circulation often develops naturally. As the fluid warms near the hotter object, its density decreases and it floats upward, lifted by the buoyant force. When the fluid cools near the colder object, its density increases and it sinks downward. Thus air heated by contact with the woodstove rises toward the ceiling and is replaced by colder air from the floor.
Eventually, this heated air cools and descends. Once it reaches the floor, it’s drawn back toward the hot woodstove to start the cycle over. This moving air is a convection current, and the looping path that it follows is a convection cell. Within the room, convection currents carry heat up and out from the woodstove to the ceiling and walls. When you put your hand over the stove, you feel this convection current rising as it transfers heat to your hand.
Natural convection is good at heating the air above the woodstove, but most of that hot air ends up near the ceiling. Although some of it will eventually drift downward to where you’re standing, convection sometimes needs help. Adding a ceiling fan will help move the hot air around the room and make the woodstove more effective. This forced convection still transfers heat from the hot stove to the colder occupants of the room, but it doesn’t rely on the buoyant force to keep the air circulating. The faster the air moves, the more heat it can transport from hot objects to cold objects.
Heat Moving as Light: Radiation
There is one more important mechanism of heat transfer—radiation. As the particles inside a material jitter about with thermal energy, they emit and absorb electromagnetic radiation. This radiation consists of electromagnetic waves, which include radio waves, microwaves, and infrared, visible, and ultraviolet light. When heat flows from a hot object to a cold object as electromagnetic radiation, we say that heat is being transferred by thermal radiation or simply radiation. Unlike conduction and convection, which depend on atoms, molecules, or electrons to carry the heat, radiation occurs directly through space. Radiative heat transfer happens even when two objects have nothing at all between them.
The types of electromagnetic waves in an object’s thermal radiation depend on its temperature. While a colder object emits only radio waves, microwaves, and infrared light, a hotter object can also emit visible or even ultraviolet light. The red glow of a hot coal in the woodstove is that coal’s thermal radiation.
Since our eyes are sensitive only to visible light, we can’t see all the thermal radiation emitted by an object, even when it’s hot. However, whether we see it or not, electromagnetic radiation contains energy and transfers heat to whatever absorbs it. While everything emits thermal radiation, the amount of that emission depends on temperature: the hotter an object, the more thermal radiation it emits. When two objects face one another, thermal radiation will travel in both directions between them. However, the hotter object will dominate this radiant exchange of thermal energy, resulting in a net transfer of thermal energy to the colder object. Exchanges of thermal energy via radiation always transfer heat from a hotter object to a colder one.
Radiation transfers a great deal of heat from the woodstove’s surface to the surrounding objects. The stove bathes the room in infrared light, which warms everything it reaches. To encourage such radiative heat transfer, the metal woodstove and its chimney are often painted black. Black not only absorbs light well, but it’s also particularly good at emitting thermal light. If you heat a black poker red hot, it will glow much more brightly than one that’s white, silvery, or transparent.
Overall, a modern woodstove is an excellent heat exchanger. As convection draws hot smoke up the long black chimney pipe, the smoke heats the stove and the pipe. These metal components conduct heat to their outer surfaces, which then distribute it around the room by convection and radiation. Although the stove consumes some room air, it controls the airflow with dampers so that it draws in only enough air to completely burn the wood. Overall, the stove extracts heat efficiently, cleanly, and safely from the burning wood.
Water, Steam, and Ice
Water is probably the most important chemical in our daily lives. It is so crucial to biology, climate, commerce, industry, and entertainment that it merits a whole section of its own. Moreover, it exhibits the three classic phases of matter—solid, liquid, and gas—and illustrates the role that heat plays in transforming one phase into another.
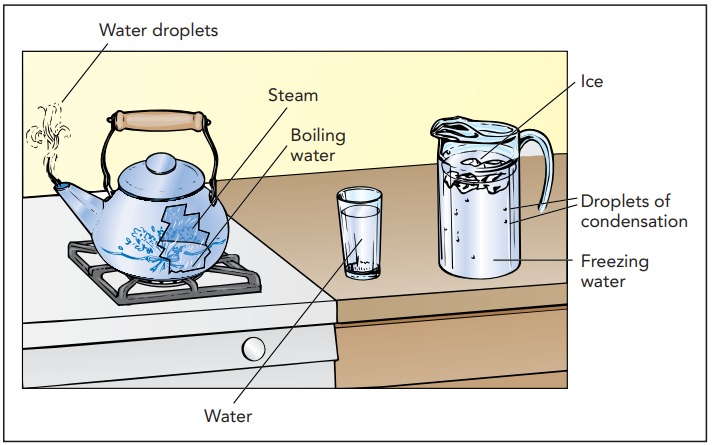
Solid, Liquid, and Gas: The Phases of Matter
Like most substances, water exists in three distinct forms or phases: solid ice, liquid water, and gaseous steam. These phases differ in how easily their shapes and volumes can change. Ice is solid, rigid and incompressible; you can’t alter an ice cube’s shape or its volume. Water is liquid, fluid but incompressible; you can reshape the water in a pitcher but you can’t change its volume. Steam is gaseous, fluid and compressible; you can vary both the shape and the volume of the steam in a tea kettle.
These different characteristics reflect the different microscopic structures of steam, water, and ice. Steam, or water vapor, is a gas, a collection of independent molecules kept in motion by thermal energy. These water molecules bounce around their container, periodically colliding with one another or with the walls. The water molecules fill the container uniformly and can accommodate any changes in its shape or size. Enlarging the container simply decreases the steam’s density and lowers its pressure.
When they are independent of one another as gaseous steam, water molecules have a substantial amount of chemical potential energy. They can release some of this energy by joining together to form ordinary water. Water is a liquid, a disorderly collection of molecules that cling to one another with chemical bonds. Because these bonds aren’t very strong, the molecules in water can use thermal energy to break them temporarily and then change bonding partners. This rebonding process allows water to change shape so that it is a fluid. Despite their flexibility, however, these bonds manage to bunch the water molecules together so snugly that even squeezing can’t pack them much tighter. That’s why water is incompressible.
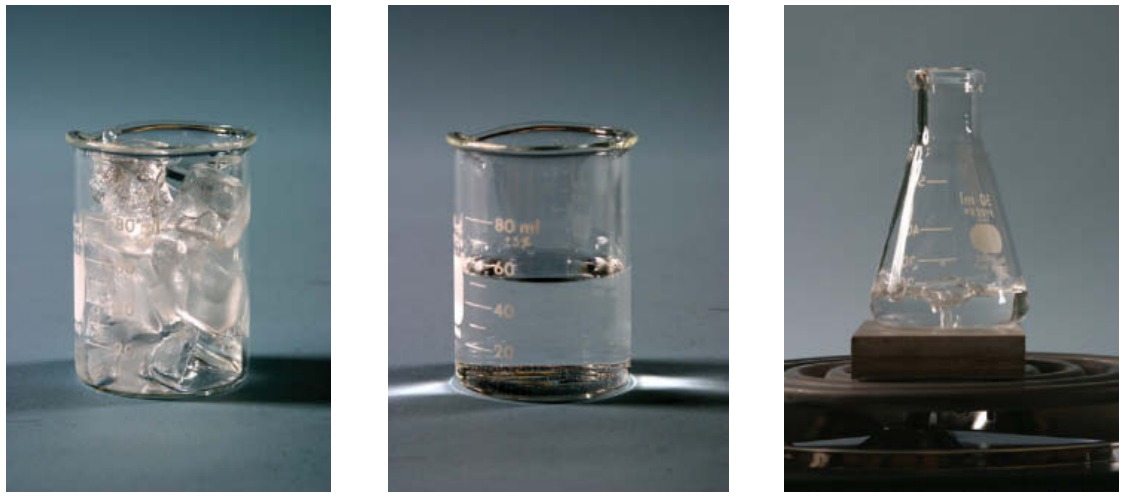
The molecules in water can release still more chemical potential energy by linking together stiffly as ice. Ice is a solid, a rigid collection of chemically bound molecules. Like most solids, ice is crystalline—its water molecules are arranged in an orderly latticework that extends over long distances and gives rise to the beautiful crystal facets seen on snowflakes and frost. Ice’s crystalline structure is so constraining that its water molecules can’t use thermal energy to change bonding partners, and consequently ice can’t change shape. Just as an orderly stack of oranges at the grocery store takes up less volume than a disorderly heap, a crystalline solid almost always occupies less volume than its corresponding disorderly liquid. The solid phase of a typical substance is thus denser than the liquid phase of that same substance, so the solid phase sinks in the liquid phase.
There is only one common substance that violates that rule—water. Ice’s crystalline structure is unusually open, and its density is surprisingly low. Almost unique in nature, solid ice is slightly less dense than liquid water, and ice therefore floats on water. That’s why icebergs float on the open ocean and why ice cubes float in your drink. In fact, water reaches its greatest density when it is liquid at about 4 °C (39 °F).
Melting Ice and Freezing Water
Ice in a freezer is extremely cold, typically −18 °C (0 °F). When you place this ice on a warm countertop, heat flows into it and its temperature rises. The ice remains solid until its temperature reaches 0 °C (32 °F). At that point, the ice stops getting warmer and begins to melt. Melting is a phase transition, a transformation from the ordered solid phase to the disordered liquid phase. This transition occurs when heat breaks some of the chemical bonds between water molecules and permits the molecules to move past one another. The melting ice transforms into water, losing its rigid shape and crystalline structure.
Zero degrees Celsius is ice’s melting temperature, the temperature at which heat goes into breaking bonds and converting ice into water, rather than into making the ice hotter. The ice-water mixture remains at 0 °C until all the ice has melted. When only water remains, heat once again causes its temperature to rise.
The heat used to transform a certain mass of solid into liquid, without changing its temperature, is called the latent heat of melting or, more formally, latent heat of fusion. The bonds between the water molecules in ice are strong enough to give ice an enormous latent heat of melting; it takes about 333,000 J of heat to convert 1 kg of ice at 0 °C into 1 kg of water at 0 °C. Since water’s specific heat is 4190 J/kg ∙ K, that same amount of heat would raise the temperature of 1 kg of liquid water by about 80 °C. Thus it takes almost as much heat to melt an ice cube as it does to warm the resulting water all the way to boiling.
The latent heat of melting reappears when you cool the water back to its melting temperature and it starts to freeze. Freezing is another phase transition, a transformation from the disordered liquid phase to the ordered solid phase. As you remove heat from water at 0 °C, the water freezes into ice rather than becoming colder. Because the water molecules release energy as they bind together to form ice crystals, the water releases heat as it freezes. The heat released when transforming a certain mass of liquid into solid, without changing its temperature, is again the latent heat of melting. You must add a certain amount of heat to ice to melt it and you must remove that same amount of heat from water to solidify it.
Phase Equilibrium: Leaving and Landing
We’ve seen that ice has a melting temperature, so now let’s see why it has a melting temperature. To do that, we must look at the interface between solid ice and liquid water. Whenever both phases are in contact, they exchange water molecules across the interface between them. Water molecules regularly break free from the ice to enter the water, and they often drop out of the water to stick to the ice. In other words, water molecules are leaving the ice and landing on it all the time, like airplanes at a busy airport.
Although you can’t see the individual leavings and landings, you can observe their net effect. If leavings outpace landings, the ice will gradually transform into water. If the landings outpace leavings, the water will gradually transform into ice. If the two processes balance one another, the ice and water will coexist indefinitely—a situation known as phase equilibrium.
Temperature plays a crucial role in this balance because it affects the rate at which water molecules leave the ice. The warmer the ice, the more often water molecules at its surface can gather enough thermal energy to break free and leave. Below ice’s melting temperature, water molecules leave the ice too infrequently to balance the landing process and the water transforms completely into ice. Above ice’s melting temperature, water molecules leave the ice so often that they outstrip the landing process and the ice transforms completely into water. Only at the melting temperature do the leaving and landing rates balance so that ice and water can coexist in phase equilibrium.
Ice’s huge latent heat of melting has a stabilizing effect on the phase equilibrium between ice and water. Whenever ice and water are mixed together, the mixture’s temperature will shift rapidly toward 0 °C. That’s because if the mixture’s temperature is above 0 °C, the ice will melt—a phase transformation that absorbs the heat of melting and thereby lowers the mixture’s temperature toward 0 °C. If the mixture’s temperature is below 0 °C, the-water will freeze—a phase transformation that releases the heat of melting and thereby raises the mixture’s temperature toward 0 °C.
As long as the mixture doesn’t run out of ice or water, its temperature will soon reach 0 °C and remain there even as you add or remove heat from it. Any heat you add to the mixture goes into melting more ice, not into raising its temperature. Any heat you remove from the mixture comes from freezing more water, not from lowering its temperature. That’s why your glass of ice water remains at 0 °C, even in the hottest or coldest weather.
Clothing, and Insulation
When you sit in front of a fire on a cold winter day, heat flows from the glowing coals to your skin and you feel warm. When you walk through the snow on your way to the store, however, heat flow is the last thing you want. As the hottest object around, you will become colder, not warmer. So you bundle up in your new down coat. Its thermal insulation keeps you warm in your frigid environment.
The Importance of Insulation
Thermal insulation slows the flow of heat from hot objects to cold and helps keep your body warm, your refrigerator cold, and your home at a comfortable temperature year round. Yet in a world where ordered energy is increasingly precious, good insulation is more than just a convenience; it’s a necessity. That’s because whenever thermal energy leaves something that must remain hot or enters something that must remain cold, replacing or removing that thermal energy consumes ordered energy. You can save ordered energy and do your part for the environment simply by using good insulation to keep thermal energy where it belongs.
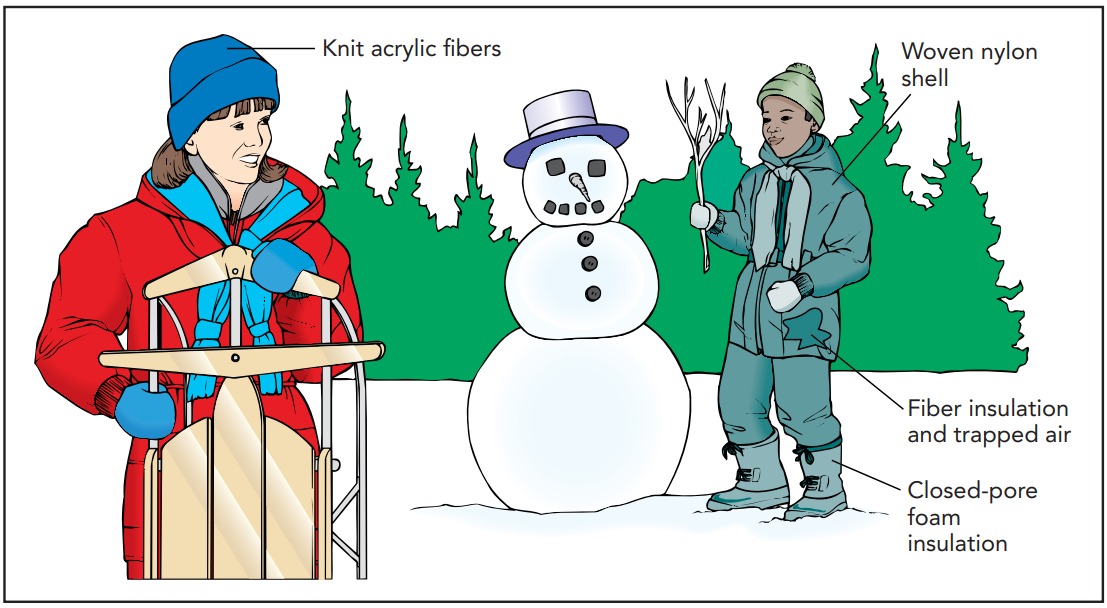
One of the most important examples of thermal insulation is your clothing. Together with your skin, clothing lowers the rate at which heat flows into or out of your body and makes it possible for you to keep your body temperature constant.
Why keep your body temperature constant? After all, cold-blooded animals such as reptiles, amphibians, and fish make no attempts to control their body temperatures. Instead, they exchange heat freely with their surroundings and are generally in thermal equilibrium with their environments. Maintaining a constant body temperature is a goal that’s unique to mammals and birds.
The chemical processes that are responsible for life are very sensitive to temperature. That sensitivity is due in part to thermal energy’s role in initiating chemical reactions; it provides the activation energies that many chemical reactions need in order to proceed. As a cold-blooded animal’s temperature decreases, there is less thermal energy per molecule and these chemical reactions occur less frequently. The animal’s whole metabolism slows down, and it becomes sluggish, dimwitted, and vulnerable to predators.
In contrast, warm-blooded animals have temperature regulation systems that allow them to maintain constant, optimal body temperatures. Regardless of its environment, a mammal or bird keeps the core of its body at a specific temperature so that it functions the same way in winter as in summer. The advantages of constant temperature are enormous. On a cold day, a warm-blooded predator can easily catch and devour its slower-moving cold-blooded prey.
There is, however, a cost to being warm-blooded. The thermal energy associated with an animal’s body temperature must come from somewhere, and the animal must struggle against its environment to maintain its temperature. Without realizing it, many of our behaviors are governed by our need to maintain body temperature. Our bodies are careful about how much thermal energy they produce, and we strive to control the rate at which we exchange heat with our surroundings.
A resting person converts chemical potential energy into thermal energy at the rate of about 80 calories per hour. Eighty calories per hour is a measure of power, equal to about 100 W, so a resting person generates about 100 W of thermal power. An active person generates even more. This steady production of thermal energy is why a room filled with people can get pretty warm. One hundred watts may not seem like much, but when a hundred people are packed into a tight space, they act like a 10,000-W space heater and the whole room becomes unpleasantly hot.
If you had no way to get rid of this thermal energy of metabolism, you would become hotter and hotter. To maintain a constant temperature, you must transfer heat to your surroundings. Since heat flows naturally from a hotter object to a colder object, your body temperature must generally be higher than the temperature around you. That requirement is one reason why human body temperature is approximately 37 °C (98.6 °F). This temperature is higher than all but the hottest locations on Earth, so heat flows naturally from your body to your surroundings. You produce thermal energy as a by-product of your activities and transfer this thermal energy as heat to your colder surroundings.
The rate at which your resting body generates thermal energy is fairly constant, so the principal way in which you stabilize your body temperature is by controlling heat loss. Like all warm-blooded animals, you have a number of physiological and behavioral techniques for doing just that. In hot weather or when you’re exercising hard, you encourage heat loss to avoid overheating. In cold weather, you limit heat loss to stay warm. Since this section is about clothing, we’re going to focus primarily on heat loss in cold weather. All three heat-transfer mechanisms are involved in that heat loss, so you must control them all to keep warm.
Staying Warm by Limiting Thermal Conduction
One way in which the body retains heat in cold weather is by impeding conductive heat loss. Heat flows through a material via conduction whenever that material is exposed to a difference in temperature—whenever one side of the material is hotter than the other side. The rate at which heat flows, however, depends on several factors: the overall temperature difference, the distance separating the hot side from the cold side, the area through which heat can flow, and the material itself.
heat flows in direct proportion to the temperature difference and to the area through which that heat can flow. Thus, if you touch the finger to two different cold surfaces, one 20 °C colder than the internal temperature and the other 40 °C colder, you’ll lose heat about twice as fast to the colder surface. If you touch two fingers at once, doubling the area through which heat can flow, you’ll lose heat about twice as fast. On the other hand, further separating the hot and cold sides slows heat flow. The rate of heat flow is inversely proportional to that distance. For example, doubling the thickness of the gloves when you’re building a snowman will double the separation between hot and cold and roughly halve the heat flow. Finally, some materials are better conductors of heat than others; they have different thermal conductivities. Skin has a particularly low thermal conductivity, meaning that it conducts relatively little heat compared to materials such as glass or copper. Thermal conductivity is a characteristic of the material itself, and its SI unit is the watt per meter-kelvin (W/m ∙ K). The overall relationship between heat flow and these four quantities can be written as a word equation:
\[ heat~ flow = \frac{thermal~ conductivity · temperature~ difference · area}{separation}\]
in symbols:
\[H = {k · \Delta T · A}{d}\]
and in everyday language:
When you pick up a hot saucepan, wear a thick oven mitt and grab a small the portion of the cooler handle
Equation tells us that the amount of heat flowing through the skin depends on its thermal conductivity, surface area, thickness, and the temperature difference across it. Amazingly, the body has optimized these factors to help you minimize heat loss. Even without clothes, you are surprisingly well insulated against conductive heat loss.
Table shows the thermal conductivities of a number of common materials.
Material | Thermal Conductivity† |
---|---|
Argon | 0.016 W/m ∙ K |
Air | 0.025 W/m ∙ K |
Oak | 0.17 W/m ∙ K |
Fat | 0.2 W/m ∙ K |
Skin | 0.21 W/m ∙ K |
Hair | 0.37 W/m ∙ K |
Brick | 0.6 W/m ∙ K |
Water | 0.6 W/m ∙ K |
Glass | 0.8 W/m ∙ K |
Marble | 2.5 W/m ∙ K |
Stainless steel | 16 W/m ∙ K |
Steel | 50 W/m ∙ K |
Aluminum | 210 W/m ∙ K |
Copper | 380 W/m ∙ K |
Silver | 429 W/m ∙ K |
Diamond | 1000 W/m ∙ K |
†The watt per meter-kelvin (abbreviated W/m ∙ K) is the SI unit of thermal conductivity
The skin and the layers immediately beneath it contain fats and other thermal insulators. Fat’s thermal conductivity is quite low, about a third that of water, so by placing fat in and just beneath the skin, the body improves its heat retention. Furthermore, the presence of a fatty layer beneath the skin effectively thickens the skin and increases the distance separating hot from cold. “Thick-skinned” people retain body heat better than those who are “thin-skinned.”
Minimizing surface area means that the body is relatively compact, shaped more like a ball than a sheet. Although other adaptive influences have given you arms, legs, and fingers, which increase the total surface area, you have little superfluous surface through which to lose heat.
Finally, the body tries to lessen conductive heat loss by reducing the temperature difference between the skin and the surrounding air. It does this by letting the hands and feet become much cooler than the core body temperature. That arrangement would be simple were it not for the circulating blood. the blood must cool down from core body temperature as it approaches the cold fingers and warm back up to core body temperature as it returns to the heart. This change in blood temperature occurs via a mechanism called countercurrent exchange. As the warm blood flows through arteries toward the cold fingers, it transfers heat to the blood returning to the heart through nearby veins. The blood heading toward the fingers becomes colder, while the blood returning to the heart becomes warmer.
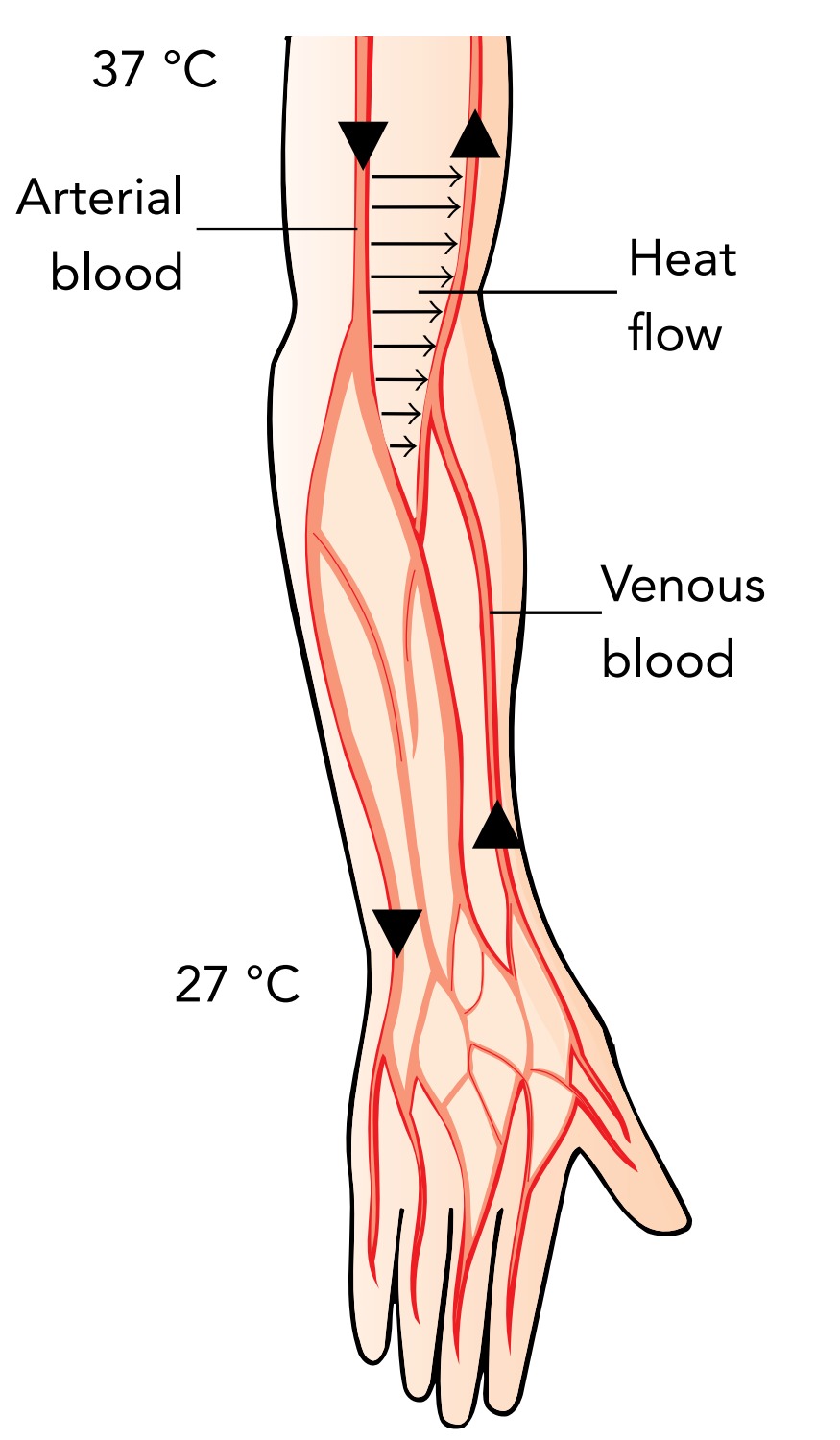
Staying Warm by Impeding Convection
Despite its great insulating qualities, skin alone isn’t enough to keep you warm in all situations. Some of the body heat inevitably flows to the surface of the skin and from there to the environment. Limiting that second step of heat flow is a job for clothing.
On a cold day, heat leaving the skin warms the nearby air. Since air is a very poor conductor of heat, however, the skin warms only a thin layer of it. If that warmed air never moved, you would have to heat it only once and that would be that. Protected by this insulating layer of air, the skin would no longer have to transport much heat and the temperature difference across the skin would shrink. You would feel warm.
But air moves all too easily. In reality, each time the skin manages to warm the nearby air, natural convection gently lifts that warmed air upward and replaces it with fresh cold air. The temperature difference across the skin thus remains large, and heat flows quickly out through the skin. You feel cold.
Wind worsens this heat loss because it blows away warmed air near the skin even faster than does natural convection. Just as a forced convection oven cooks food faster, so a forced convection freezer (such as a cold, windy day) chills people faster. The enhanced heat loss caused by moving air is called wind chill—you feel even colder on a windy day.
To combat convective heat loss and wind chill, most warm-blooded animals are covered with hair or feathers. These finely structured materials are poor conductors of heat, but their main purpose is actually to trap an even better insulator: air. In the dense tangle of a sheep’s wool, air experiences such severe drag forces that it can barely move at all. Since convection requires airflow, the sheep can lose heat only via conduction through the trapped air and wool. That combination of materials is a terrible conductor of heat, so the sheep stays warm.
We humans have relatively little hair and are thus poorly adapted to living in cold, windy climates. Our lack of natural insulation is one of the reasons we wear clothing. Like hair and feathers, our clothing traps the air and reduces convection. Finely divided strands or filaments are particularly effective at stopping the flow of air. Not surprisingly, the best insulating clothing is made of hair (natural or synthetic) and feathers (also natural or synthetic). Since motionless air has a lower thermal conductivity than any of the materials that trap it, the ideal coat uses only enough material to keep a thick layer of air from moving. This discussion also applies to swimming in cold water. If the water near the skin would stay put, you could warm up a layer of it and then feel relatively warm. That’s why some swimmers wear wet suits. The spongy material in a wet suit keeps the layer of water near the swimmer’s skin from moving. As long it remains in place, water is a respectable thermal insulator.
Absorbing Thermal Radiation
When you sit in front of a glowing fire, you feel its thermal radiation warming the skin. But the skin also emits thermal radiation, so what you’re really noticing is that the skin is absorbing more thermal radiation from the fire than it’s emitting toward the surroundings. You’re gaining heat. In contrast, if you sit inside an ice sculpture castle, the skin will absorb less thermal radiation from the ice than it’s emitting, so you’ll be losing heat and feeling cold.
One can often judge the temperature of a hot object by the color of the light it emits. An object that’s glowing red is too hot to touch, but one that’s glowing yellow-white is a serious fire hazard. That connection between temperature and color isn’t a coincidence. The light that you see emanating from a hot object is part of that object’s thermal radiation. You are quite literally seeing its heat.
However, you’re not seeing all of its heat. Although thermal radiation consists of electromagnetic waves and you can see some electromagnetic waves, most are invisible to the eyes. Those that you can see, visible light, are part of a continuous spectrum of electromagnetic radiation that extends from radio waves at one e
an object’s thermal radiation isn’t a single electromagnetic wave with one specific wavelength. Instead, it’s many individual waves that cover a broad range of wavelengths. The distribution of those wavelengths depends on the object’s temperature and surface properties, particularly its emissivity, the efficiency with which it emits and absorbs thermal radiation. Emissivity is measured on a scale from 0 to 1, with 1 being ideal effi- ciency. A perfectly black object has an emissivity of 1; it absorbs all light that strikes it and emits thermal light as efficiently as possible.
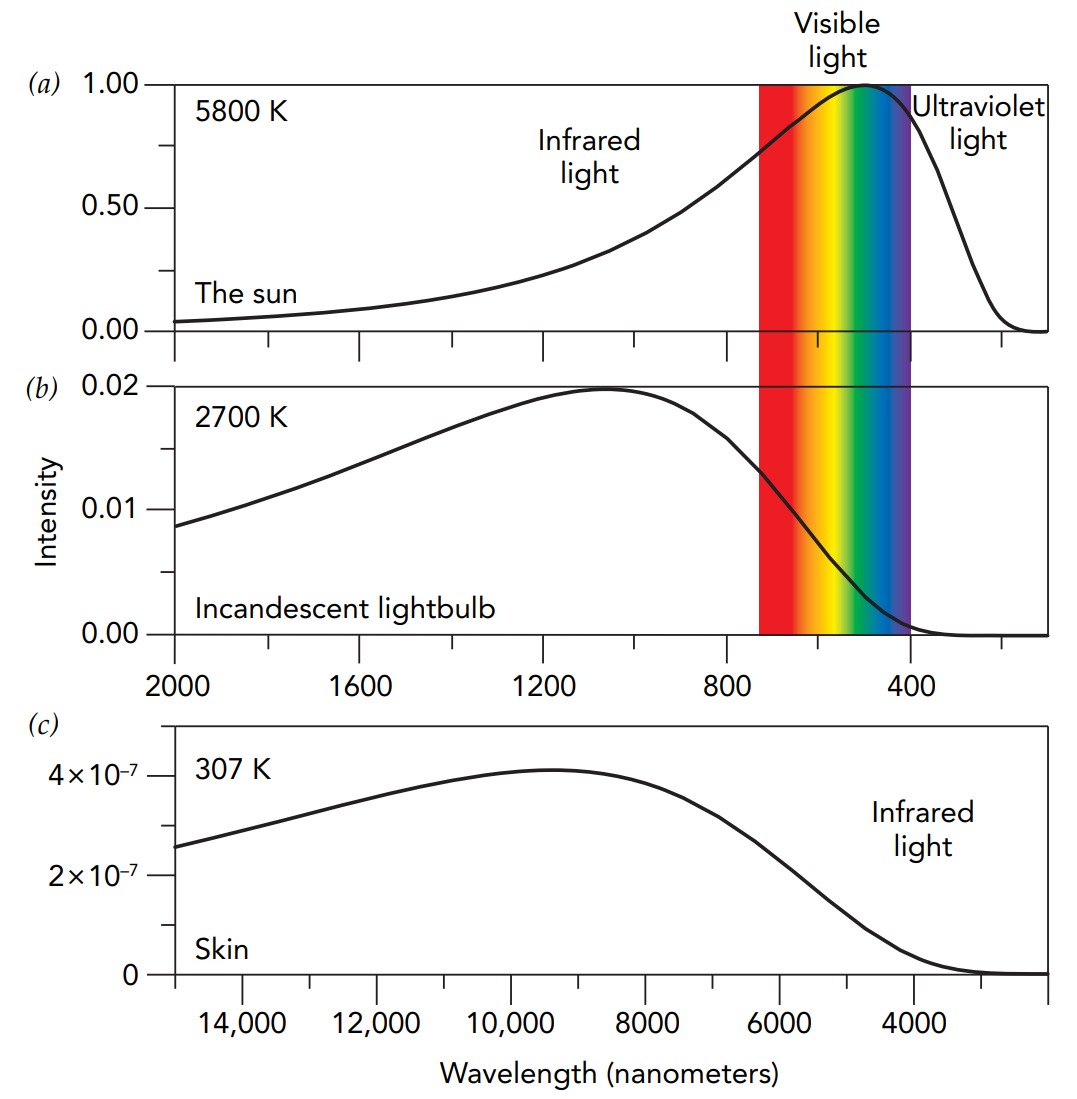
The distribution of wavelengths emitted by a black object is determined by its temperature alone and is called a blackbody spectrum. The spectrum brightens and shifts toward shorter wavelengths as the object’s temperature increases. When a black object is colder than about 400 °C, its thermal radiation lies entirely in the invisible long-wavelength portion of the spectrum and you can’t see the radiation at all. Even at 400 °C, only the night vision is able to sense the thermal radiation, which appears dim and colorless. At about 500 °C, the color vision begins to detect the glow and the eyes make an average assessment of the distribution of wavelengths. You see dull red. With increasing temperature, you then observe reddish, orangish, yellowish, whitish, and eventually bluish light. The temperature of thermal radiation associated with a particular distribution of wavelengths is the color temperature of that light.
Whether visible or not, the entire spectrum of thermal radiation is important to retaining body heat. the body radiates thermal power and if the radiated power is greater than the thermal power you absorb from the environment, you are losing heat via radiation.
The thermal power you radiate depends primarily on the temperature. It’s clear from Fig. that a black object radiates more power as its temperature increases. What may be surprising, however, is how dramatically that radiated power increases with temperature: it is proportional to the fourth power of the absolute temperature! Thus, although a black pot full of boiling water (373 K) and an identical black pot full of ice water (273 K) have an absolute temperature ratio of only 1.37, the hotter one radiates 1.374 , or 3.5, times as much thermal power as the cooler one.
Object | Temperature | Color |
---|---|---|
Skin | 34 ºC (93 ºF) | Invisible |
Heat lamp | 500 ºC (930 ºF) | Dull red |
Candle flame | 1700 ºC (3100 ºF) | Dim orange |
Incandescent bulb | 2500 ºC (4500 ºF) | Bright yellow-white |
Sun’s surface | 5525 ºC (9980 ºF) | Brilliant white |
Blue star | 6000 ºC (10,800 ºF) | Dazzling blue-white |
The radiated power also depends on the surface area and on the emissivity, and it is proportional to each of those quantities. The precise relationship between temperature and radiated power can be written as a word equation:
\[ radiated ~power = emissivity · Stefan–Boltzmann~ constant · temperature^{4} · surface ~area\]
in symbols:
\[P = e ∙ \sigma ∙ T^{4} ∙ A\]
and in everyday language:
Hot food radiates away lots of heat, so wrap it compactly in aluminum foil to keep it warm
This relationship is called the Stefan–Boltzmann law, and the Stefan–Boltzmann constant (σ) that appears in it has a measured value of \(5.67 \times 10^{−8}\) J/(s ∙ m\(^{2}\) ∙ K\(^{4}\)). The temperature must be measured in kelvins.
The skin’s temperature is only 307 K, however, so its thermal radiation depends on its low-temperature emissivityregardless of the appearance in visible light, the skin is almost perfectly black at the infrared wavelengths of skin-temperature thermal radiation. The skin’s low-temperature emissivity is approximately 0.97. In fact, most nonmetallic objects have low-temperature emissivities greater than 0.95. If you could see infrared light rather than visible light, all the people and most of the objects around you would look black—you would see that they absorb almost all the infrared light that strikes them and that they glow brightly with their own infrared thermal radiation.
Staying Warm by Controlling Thermal Radiation
The average temperature of human skin is about 307 K (34 °C or 93 °F), and a typical person has a body surface area of about 1.8 m\(^2\( (19 ft\(^2\)). Assuming an emissivity of 0.97, the Stefan–Boltzmann law predicts that a person will radiate about 850 W of thermal power. If you actually lost heat that fast, you’d cool off quickly and be very uncomfortable. Thus, limiting radiative heat loss is important to maintaining your body temperature.
Fortunately, everything around you is also emitting thermal radiation, and you typically absorb about as much thermal power as you emit. You even absorb some of your own thermal radiation. If you hold your hand in front of your face on a cold day, you’ll feel its thermal radiation warming your face.
However, whenever there’s a temperature difference between you and your environment, heat will flow from hotter to colder every way it can, including radiation. While conduction and convection transfer heat in proportion to the difference in temperature, radiation transfers heat in proportion to the difference between the fourth powers of the absolute temperatures. That exotic relationship between temperatures and heat flow explains why radiative heat transfer to or from your skin is most noticeable when you’re exposed to unusually hot or cold objects.
For example, the sun warms your skin quickly because it radiates more heat at you than the rest of your surroundings combined. Measured on an absolute temperature scale, the sun’s surface temperature (5800 K) is about 20 times that of your skin (307 K). Though it’s very distant and appears small to your eye, the sun radiates about 204, or 160,000, times as much heat at you as you radiate at it.
In contrast, the dark night sky cools you quickly because it’s actually colder than your surroundings. The nearly empty space beyond Earth’s atmosphere is only a few degrees above absolute zero, and even with the atmosphere’s considerable help, the sky’s effective radiant temperature is still about 20 °C colder than the air around you. When you lie on the snow in an open field and look up at the clear night sky, your 307-K skin radiates about twice as much heat at the approximately 250-K sky as it radiates back at you. You lose heat quickly and feel cold.
You can improve your situation by moving under a sheltering pine tree. Although the tree is no warmer than the snow, it’s about 20 °C hotter than the sky and therefore emits more thermal radiation at you. Although the tree isn’t quite a crackling campfire, it will still help to keep you warm.
That said, we still have you running back and forth between hot and cold objects to keep warm. It’s time to look at how you can control thermal radiation while staying in one place.
You can start by wearing a heavy coat. The coat’s internal insulation allows its outer surface to cool down to the temperature of your environment, so that it radiates only a little more thermal power than it absorbs and doesn’t lose much heat. This strategy is common in birds and mammals. Penguins and polar bears let the outsides of their insulated feathers and fur cool to the temperatures of their frozen worlds and therefore lose almost no heat by radiation.
You can also change your emissivity to control radiative heat transfer. We’ve seen that black surfaces are ideal whenever you want to exchange thermal radiation with other objects, but what about when you don’t want to exchange thermal energy? That’s where shiny, white, and transparent surfaces come into play.
A perfectly shiny or white surface has an emissivity of zero; it reflects all of the light that strikes it, absorbing none, and is itself unable to emit any thermal radiation. If you put a shiny or white surface between yourself and a snowman, you’ll each see your own thermal radiation coming back at you and you won’t exchange any heat. This insulating effect works even if one of you wears the shiny or white surface as clothing; whoever is wearing it won’t emit thermal radiation at all, and the other will see his or her own thermal radiation returned by that clothing.
Unfortunately, while truly white clothing would make you immune to radiant heat transfer, such clothing doesn’t exist. Clothing exemplifies the difference between hightemperature and low-temperature emissivities. Regardless of their colors in visible light, virtually all clothing materials are black to infrared light and have low-temperature emissivities close to 1. In other words, most clothes absorb and emit low-temperature thermal radiation almost perfectly. Thus, although wearing a white robe on a scorching summer day will reduce the amount of thermal radiation you absorb directly from the sun because the robe’s high-temperature emissivity is nearly 0, it won’t protect you from the sun-warmed objects around you because its low-temperature emissivity is nearly 1.
In contrast, shiny clothing can protect you from those sun-warmed objects, but only if it contains metals. Metals have small low-temperature emissivities because they conduct electricity, a property that allows them to reflect electromagnetic waves extremely well. When you wrap food in aluminum foil, its emissivity drops to about 0.05 and it can barely exchange heat by radiation. Wearing metallic clothes has the same effect for people. Lamé fabrics—fabrics with metal threads woven into them—help to keep their typically underdressed wearers warm. A less flamboyant use of metallic cloth is in the-metal-coated plastic blankets that are part of emergency rescue kits; wrapping yourself in one of these blankets shiny-side out keeps you from exchanging thermal radiation with your surroundings.
Smoke jumpers who battle forest fires occasionally get trapped by the fires they’re fighting and must try to survive as the fires burn over them. Their chances improve significantly if they use small personal survival shelters that have shiny metallic surfaces. Huddled against the cool ground underneath one of these tent-like shelters, a firefighter is relatively insulated from the fire overhead. Assuming the firefighter is in a low spot with nothing to burn nearby and makes no contact with the shelter itself, conduction and convection can’t convey much heat to the firefighter. And with the shelter’s shiny, low-emissivity surface reflecting most of the fire’s thermal radiation, radiative heat transfer is also greatly diminished. On August 29, 1985, 73 firefighters were trapped for several hours by a fire in the Salmon National Forrest near Salmon, Idaho, and survived only with the aid of their personal fire shelters.
Transparent materials also have emissivities near 0 and neither absorb nor emit thermal radiation well. Unlike shiny or white surfaces, however, transparent materials avoid absorbing thermal radiation by letting it pass through them. For example, when sunlight streams through a window to warm your skin, it’s obvious that glass doesn’t absorb much of the sun’s thermal radiation. As for emission, any artist who sculpts molten glass knows from painful experience that glass provides almost no visible warning that it’s extremely hot.
However, emissivity once again varies with temperature. Nearly all the materials that are transparent to the visible light of high-temperature thermal radiation, including glass, are black to the infrared light of low-temperature thermal radiation. That’s probably just as well for clothing because clothing that’s transparent to thermal radiation wouldn’t provide much insulation.
Insulating Houses
The goal of housing insulation is to limit heat flow into or out of a house so that the temperature inside the house is nearly independent of the temperature outside. It’s based on the same insulating concepts that help keep people and animals warm, but because houses and their contents rarely move, they can employ insulating methods and materials that are heavy, bulky, rigid, or fragile.
While there are many solid materials that are poor conductors of heat, including glass, plastic, wood, sand, and brick, they aren’t nearly as insulating as air. Of course, since air tends to undergo convection, it needs to be held in place by porous or fibrous materials such as glass wool, sawdust, plastic foam, or narrow channels. Trapped air is the primary insulation in most buildings.
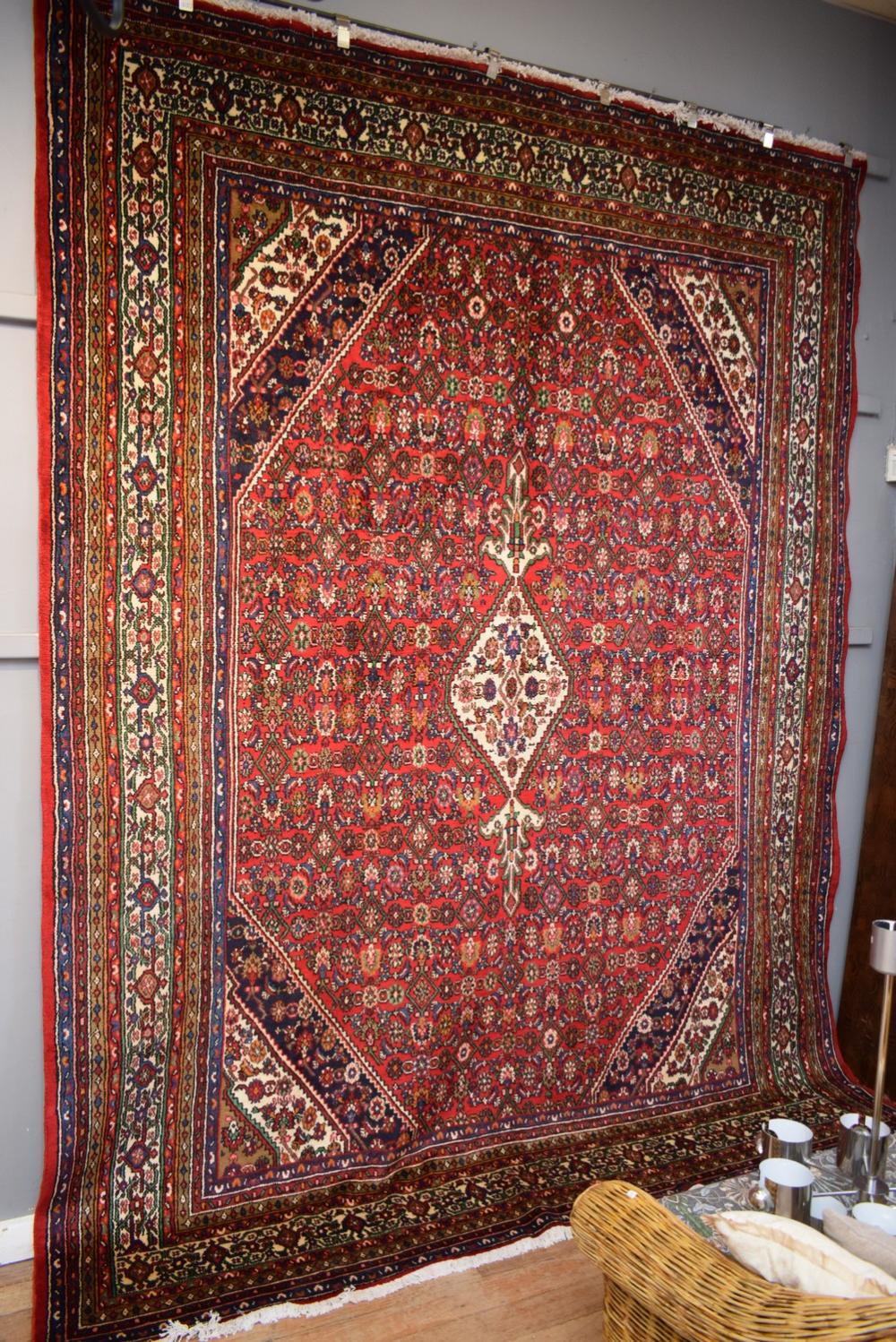
Glass wool or fiberglass is made by spinning molten glass into long, thin fibers that are then matted together like cotton candy. Solid glass is already a poor conductor of heat, but reducing it to fibers makes it even more insulating. The path that heat must take as it’s conducted through the tangled fibers is so long and circuitous that little heat gets through. Most of the volume in glass wool is taken up by trapped air. The glass fibers keep the air from undergoing convection so the air can carry heat only by conduction. Together, glass wool and the air trapped in it make excellent insulation. Since they’re also nonflammable, they are commonly used in ovens, hot-water heaters, and other hightemperature appliances. Most modern houses have about 10–20 cm (4–8 in) of glass wool insulation built into their outside walls, along with a vapor barrier to keep the wind from blowing air directly through the insulation.
Although stone is not a good conductor of heat, it’s not nearly as good an insulator as air trapped in a fibrous mat. Medieval stone castles were notoriously cold in winter because heat flowed too easily out of them through their stone walls. This tapestry slows the flow of heat to the outside air and helps to keep the room warm.
Because hot air rises and cold air sinks, the temperature difference between the hot air below the ceiling of the top floor and the cold air above that ceiling can become quite large during the winter. That ceiling is thus a very important site of unwanted heat transfer and requires heavy insulation. Glass wool inserted above the ceiling of the top floor in a modern house may be more than 30 cm (12 in) thick.
While glass wool is an excellent insulator, other materials are used in certain situations. Urethane foam and polystyrene foam are waterproof, windproof, and even better insulators than glass wool. Unfortunately, they’re also flammable and fragile. Nonetheless, they are frequently used in buildings and in refrigerators and freezers, where condensation makes waterproof insulation a necessity. Polystyrene foam also makes insulating cups that keep hot coffee hot and cold drinks cold. Unfortunately, polystyrene foam is hard to recycle, so many coffee shops now use alternatives that are less insulating but more environmentally friendly.
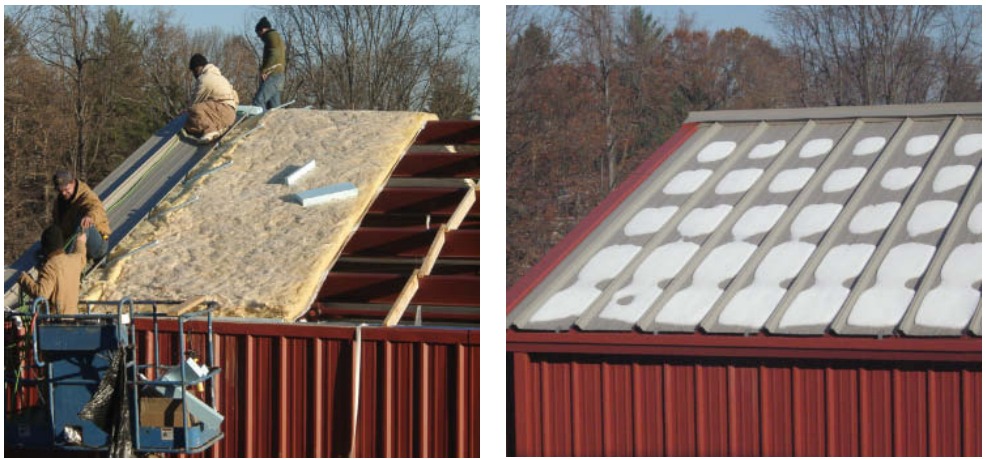
Modern Insulating Windows
Since windows have to be transparent, they can’t be filled with fiberglass or foam, and a window made of solid glass is a poor insulator unless it’s extremely thick. Insulating windows requires a different approach. The most common way to insulate a window is to use two thin panes of glass that are spaced a centimeter or two apart. That vertical gap is typically filled with argon gas, which is an even poorer conductor of heat than air. Although convection occurs inside the gap, the convection cells that form are tall and thin and therefore relatively ineffective at carrying heat from one pane to the other. When properly designed, assembled, and installed, a double-pane window transports relatively little heat by conduction or convection.
Although ordinary glass is clear to visible light, it has a low-temperature emissivity of 0.92 and is nearly black to long-wavelength infrared light. Thus the glass panes of a conventional double-pane window are exchanging thermal radiation with almost perfect efficiency. If there’s a big temperature difference between the inside and outside panes, heat will flow via thermal radiation from the hotter pane to the colder pane and spoil the window’s insulating behavior.
To decrease this radiant heat flow, an energy-efficient low-emissivity (Low-E) window has a thin coating applied to the inner surface of one of its panes. That coating makes the pane’s surface behave like a shiny mirror for infrared light; it emits very little thermal radiation, and it reflects back most of what it receives from the other pane. The coating doesn’t reflect visible light, though, so the window still looks clear. Known as a heat mirror because it reflects only low-temperature thermal radiation, this coating dramatically reduces the flow of radiant heat through the window.
One of the most common Low-E coatings is indium-tin-oxide, a transparent electrical conductor that’s also widely used in electronic displays for watches and computers. Like a metal, indium-tin-oxide uses its electrical conductivity to reflect electromagnetic waves. It has a low-temperature emissivity of about 0.10, so it’s almost as reflective as aluminum foil. However, because indium-tin-oxide’s conductivity is limited, it can respond only to long-wavelength electromagnetic waves. It reflects low-temperature thermal radiation but transmits visible light.
By suppressing all three heat transfer mechanisms, a Low-E window is able to provide surprisingly good insulation. The temperature of its outer pane matches the outdoor temperature and the temperature of its inner pane matches the indoor temperature, yet little heat flows through the gap between those panes.
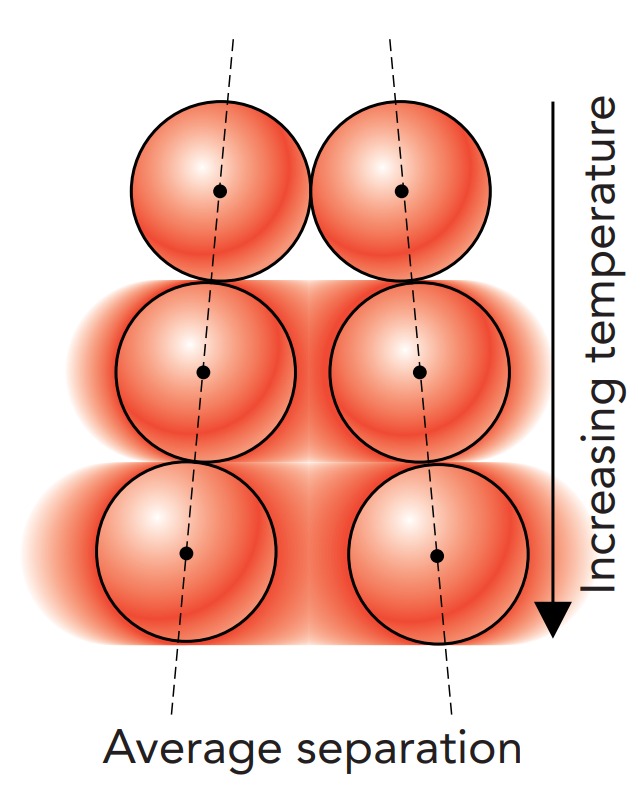
One potential problem for Low-E windows is leakage. If the argon gas escapes from between the two panes and is replaced by moist air, the heat mirror coating may degrade and the window can become foggy. Keeping the window assembly perfectly sealed for decades is a challenge, particularly when that assembly is subject to large changes in temperature. The metals, plastics, and glass used in the window assembly don’t all expand equally as they warm up, and the assembly can literally tear itself apart.
A material’s thermal expansion is caused by atomic vibrations. Because of thermal energy, adjacent atoms vibrate back and forth about their equilibrium separations (Fig. 7.3.8). This vibrational motion isn’t symmetrical; the repulsive forces the atoms experience when they’re too close together are stiffer than the attractive forces they experience when they’re too far apart. As a result of this asymmetry, they push apart more quickly than they draw together and thus spend most of their time at more than their equilibrium separation. On average, their actual separation is larger than their equilibrium separation, and the material containing them is bigger than it would be without thermal energy.
As an object’s temperature rises, it grows larger in all directions. The extent to which it expands with increasing temperature is normally described by its coefficient of volume expansion, the fractional change in the object’s volume per unit of temperature increase. Fractional change in volume is the net change in volume divided by the total volume. Since most materials expand only a small amount when becoming 1 °C (or 1 K) hotter, coeffi- cients of volume expansion are small, typically about 2.5 \(\times 10^{−5}\) \(K^{−1}\) for glass, 5 \( \times 10^{−5}\) \(K^{−1}\) for metals, and 2 \(\times 10^{−4}\) \(K^{−1}\) for plastics.
As the window assembly heats up, its plastics expand more than its metals, which expand more than its glass. Those differences in expansion put stresses on the components and can separate them or break them. Making an assembly that tolerates thousands of warming and cooling cycles without leaking is a big deal.