Heat normally flows from a hotter object to a colder object, which is why the hot sun warms your skin as you sit on a beach and why a cold winter breeze cools it as you sled down a mountain. But not everything in nature permits heat to flow passively. Our technological world includes many devices that actively transfer heat from colder objects to hotter objects or that use the flow of heat to do useful work.
Air Conditioners
On a summer day, your problem isn’t staying warm; it’s keeping cool. Instead of looking for something to burn in your woodstove, you turn on your air conditioner. An air conditioner is a device that cools room air by removing some of its thermal energy. The air conditioner, however, can’t make thermal energy either disappear or become ordered energy. Instead, it transfers thermal energy from the cooler room air to the warmer air outside. Since the air conditioner transfers heat against its natural direction of flow, the air conditioner is a heat pump.
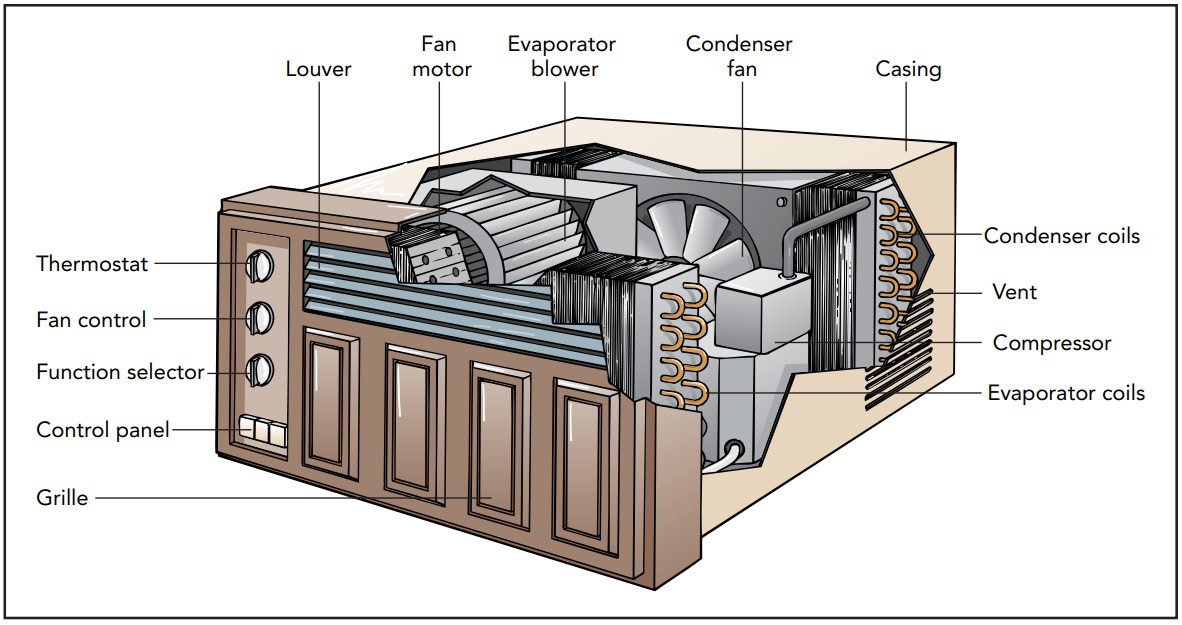
Moving Heat Around: Thermodynamics
On a sweltering summer day, the air in your home becomes unpleasantly hot. Heat enters your home from outdoors and doesn’t stop flowing until it’s as hot inside as it is outside. You can make your home more comfortable by getting rid of some of its thermal energy. Although we’ve already looked at ways to add thermal energy to room air, we haven’t yet learned how to remove it. At present, the only cooling method we’ve discussed is contact with a colder object. Unless you have an icehouse nearby, you need another scheme for eliminating thermal energy. You need an air conditioner.
An air conditioner transfers heat against its natural direction of flow. Heat moves from the colder air in your home to the hotter air outside so that your home gets colder while the outdoor air gets hotter. There’s a cost to transferring heat in this manner. The air conditioner requires ordered energy to operate and typically consumes large amounts of electric energy. It’s a type of heat pump, a device that uses ordered energy to transfer heat from a colder object to a hotter object, against its natural direction of flow.
Before studying how an air conditioner pumps heat, we should first show that pumping is necessary. There are three harebrained cooling alternatives that we need to discredit before turning to air conditioning:
- Letting heat flow from your home to your neighbor’s home.
- Destroying some of your home’s thermal energy.
- Converting some of your home’s thermal energy into electric energy.
This will help us in understanding the laws governing the movement of thermal energy, the laws of thermodynamics.
Your home is in thermal equilibrium with the outdoor air, meaning that no heat flows from one to the other and they’re at the same temperature. Your neighbor’s home is also in thermal equilibrium with the outdoor air. What will happen if you permit heat to flow between your home and your neighbor’s home? Nothing. Since both homes are simultaneously in thermal equilibrium with the outdoor air, they’re also in thermal equilibrium with one another. All three are at the same temperature.
This observation is an example of the law of thermal equilibrium (often called the zeroth law of thermodynamics), which says that two objects that are each in thermal equilibrium with a third object are also in thermal equilibrium with one another.
This seemingly obvious law is the basis for a meaningful system of temperatures. If you had a roomful of objects at 35 °C (95 °F) and some were in thermal equilibrium with one another while others were not, then “being at 35 °C” wouldn’t mean much. However, every object that has a temperature of 35 °C is in thermal equilibrium with every other object at 35 °C. The law of thermal equilibrium is observed to be true in nature, so temperature does have meaning. Since your neighbor’s home is just as hot as yours, they can relax because you’re not going to be sending them any extra heat.
THE LAW OF THERMAL EQUILIBRIUM
Two objects that are each in thermal equilibrium with a third object are also in thermal equilibrium with one another.
The second alternative sounds unlikely from the outset. You can’t cool your home by destroying thermal energy because energy can’t be destroyed. To eliminate thermal energy, you must convert it to another form or transfer it elsewhere. This concept of energy conservation is the basis for the law of conservation of energy (often called the first law of thermodynamics), which officially recognizes that there are actually two ways to transfer energy: the mechanical means we know as work and the thermal means we know as heat. You can increase an object’s internal energy, which includes its thermal energy, only by doing work on it or by transferring heat to it
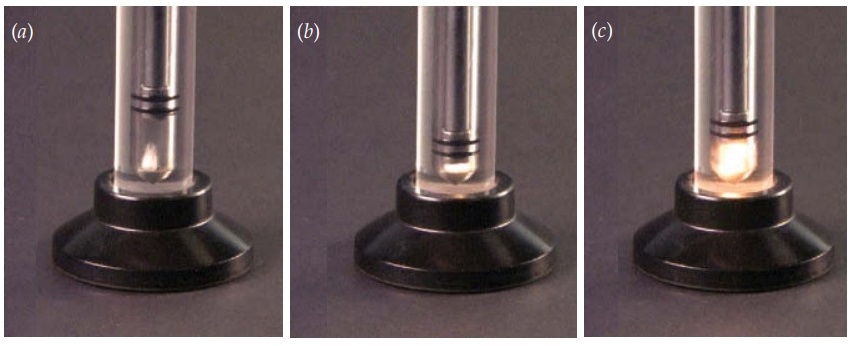
The law of conservation of energy observes that the change in a stationary object’s internal energy is equal to the heat transferred into that object minus the work that object does on its surroundings. In other words, heat added to the object increases its internal energy, while work done by the object decreases its internal energy. The law of conservation of energy can be written as a word equation:
\[change~ in ~object’s ~internal~ energy = heat~ added~ to~ object − work ~done~ by ~object\]
in symbols,
\[\Delta U = Q − W\]
and in everyday language:
You can add energy to a ball as heat by cooking it or as work by squeezing it.
THE LAW OF CONSERVATION OF ENERGY
The change in a stationary object’s internal energy is equal to the heat transferred into that object minus the work that object does on its surroundings.
Disorder and Entropy
The third alternative looks much more promising than the first two. It seems as though you should be able to convert thermal energy into electricity (or some other ordered form of energy). You could then sell it back to the electric company and get credit on your bill. Wouldn’t that be great? There’s a problem with this idea—ordered energy and thermal energy aren’t equivalent. You can easily convert ordered energy into thermal energy, but the reverse is much harder.
For example, you can burn a log to convert its chemical potential energy into thermal energy, but you’ll have trouble converting that thermal energy back into chemical potential energy to recreate the log.
The basic laws of motion are silent on this issue. The log’s original energy and constituent particles still exist, and they could, in principle, act together to reassemble the log. It is the laws of statistics that prevent the log’s reassembly. That reassembly process would require a long series of remarkably unlikely events. The particles would all have to move in just the right ways to turn the burned gases back into wood and oxygen, an incredible coincidence that simply never happens. Similarly, all the air particles in your home would have to act together to convert their thermal energy into electricity. Since that coordinated behavior is ridiculously improbable, you’re not going to be selling thermal-energy power to the electric company any time soon.
Once ordered energy has been scattered randomly among the individual air particles, you can’t collect that energy back together again. Creating disorder out of order is easy, but recovering order from disorder is nearly impossible. As a result, systems that begin with some amount of order gradually become more and more disordered, never the other way around. The best they can do is to stay the same for a while so that their disorder doesn’t change. From these observations, we can state that the disorder of an isolated system never decreases.
This notion of never-decreasing disorder is one of the central concepts of thermal physics. There is even a formal measure of the total disorder in an object, entropy. All disorder contributes to an object’s entropy, including its thermal energy and its structural defects. Both breaking a window and heating it increase its entropy. Although its name sounds similar to energy, don’t confuse energy and entropy. Energy is a conserved quantity, while entropy is a quantity that can and generally does increase. It’s easy to make more entropy.
Because disorder never decreases, the third cooling alternative is impossible. Turning your home’s thermal energy into electric energy would reduce its disorder and decrease its entropy. Our observations about entropy aren’t yet complete, though. There is one way to decrease your home’s entropy: you can export that entropy somewhere else. In fact, you export entropy every time you take out the garbage, though that action also changes the contents of your home. You can also export entropy without modifying your home’s contents by transferring heat somewhere else. Heat carries disorder and entropy with it, so getting rid of heat also gets rid of entropy.
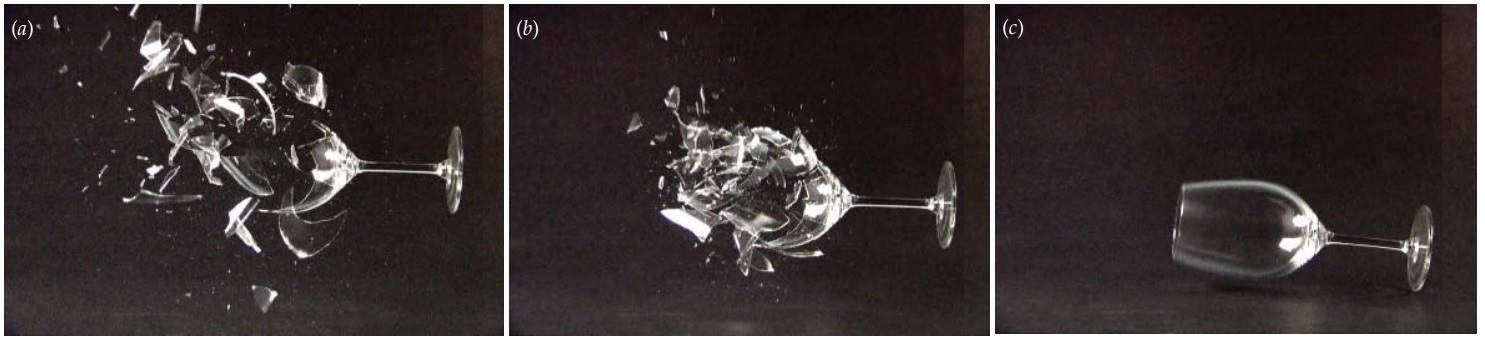
Our rule about entropy never decreasing is weakened by the possibility of exchanging heat and entropy between objects. Before asserting that an object or system of objects can’t decrease its entropy, we must ensure that the object is thermally isolated from its surroundings so that it can’t export its entropy. With that in mind, the strongest statement that we can make concerning entropy is that the entropy of a thermally isolated system of objects never decreases. This observation is the law of entropy (often called the second law of thermodynamics).
THE LAW OF ENTROPY
The entropy of a thermally isolated system of objects never decreases.
Because of the law of entropy, the only way to cool your home is to export its thermal energy and entropy elsewhere. Such a transfer would be easy if you had a cold object nearby to receive the heat. Lacking a cold object, you must use an air conditioner. Like all heat pumps, an air conditioner transfers heat and entropy in such a way that the law of entropy is never violated and the entropy of each thermally isolated system of objects never decreases. The air conditioner lowers the entropy of your home but raises the entropy of the outdoor air even more, so that, overall, the entropy of the world actually increases.*
Pumping Heat Against Its Natural Flow
Although the law of entropy doesn’t allow the entropy of a thermally isolated system to decrease, it does permit the objects in that system to redistribute their individual entropies. One object’s entropy can decrease as long as the entropy of the rest of the system increases by at least as much. Such entropy redistribution allows part of the system to become colder if the rest of the system becomes hotter.
For example, suppose that there’s a pond of cold water behind your home. You pump that water through your bathtub and let it draw heat out of the room air. Your home becomes colder while the pond becomes warmer. This transfer of heat from the hot air in your home to the cold water in the pond satisfies the law of entropy. The entropy of the combined system—your home and the pool of water—doesn’t decrease. In fact, it actually increases! This entropy increase occurs because heat is more disordering to cold objects than it is to hot objects. Each joule of heat that flows from your home to the pool creates more disorder in the pool than it creates order in your home.
A useful analog for this effect involves two parties taking place simultaneously: the garden society’s annual tea party and a 4-year-old’s birthday party. The orderly tea party represents the cold pool while the disorderly birthday party represents your hot home. The analogy to letting heat flow from your hot home to the cold pool is to trade one lively 4-year-old from the disorderly birthday party for one quiet octogenarian from the orderly tea party. This exchange will reduce the birthday party’s disorder only slightly, but it will dramatically increase the disorder of the tea party. The attendance at each party will be unchanged but their total disorder will increase. When heat flows from your home to the pool, the overall entropy increases and the law of entropy is more than satisfied. A similar increase in entropy occurs whenever heat flows from a hot object to a cold object, which is why heat normally flows in that direction.
An air conditioner, however, does the seemingly impossible: it transfers heat from a cold object, your home, to a hot object, the outdoor air. This heat flows in the wrong direction, and the disorder it creates by entering the hot outdoor air is less than the disorder it removes by leaving the cold indoor air. It’s like returning the tea party’s lone 4-year-old to the birthday party in exchange for the elderly garden fancier—the birthday party becomes only a tiny bit more disorderly while the garden party becomes much more orderly, so the net disorder of the two gatherings decreases substantially. Similarly, if nothing else happened when the air conditioner moved heat from the cold indoor air to the hot outdoor air, the entropy of the combined system would decrease and the law of entropy would be violated!
However, we’ve omitted an important feature of the air conditioner’s operation—the electric energy it consumes. The air conditioner converts this ordered energy into thermal energy and delivers it as additional heat to the outdoor air. In doing so, the air conditioner creates enough extra entropy to ensure that the overall entropy of the combined system increases. The law of entropy is satisfied after all
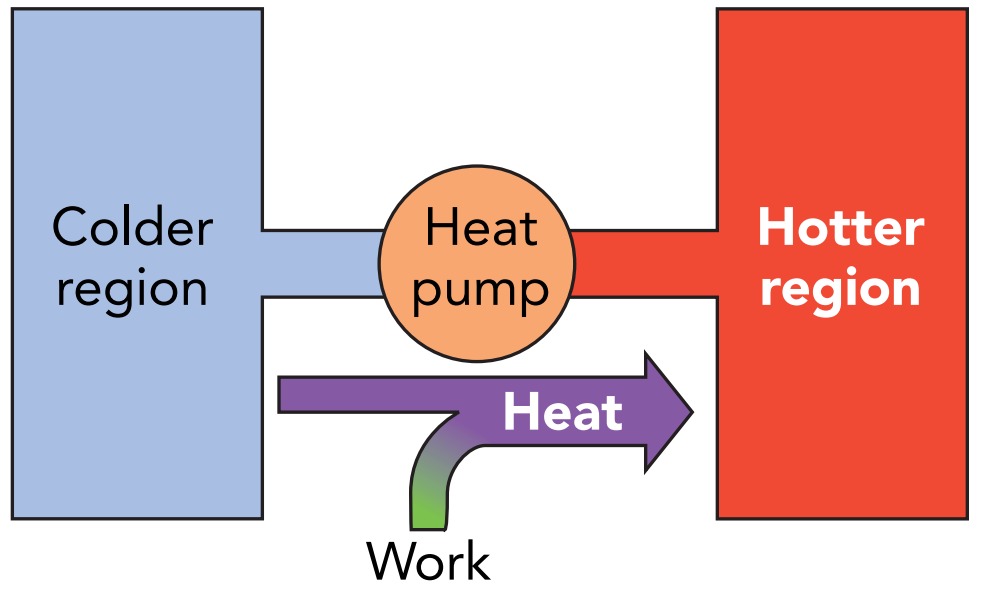
The amount of ordered energy the air conditioner consumes depends on the temperatures of the indoor air and outdoor air. If the two are close in temperature, the transfer of heat reduces the entropy only slightly, so the air conditioner doesn’t need to convert much ordered energy into thermal energy. If they are far apart in temperature, however, the air conditioner must create lots of extra entropy to make up for the entropy lost in the transfer.
This requirement that entropy not decrease explains why an air conditioner works best when it’s cooling your home the least. The greater the temperature difference between the indoor air and the outdoor air, the more electric energy or other form of work the air conditioner must consume to transfer each joule of heat. For an ideally efficient air conditioner or other heat pump, the relationships between the work consumed, the heat removed from the cold object, and the heat added to the hot object can be written as two word equations:
\[heat ~removed~ from ~cold ~object = work ~consumed · \frac{temperature_{cold}}{temperature_{hot} − temperature_{cold}} \]
\[heat~ added ~to~ hot ~object = heat~ removed ~from ~cold ~object + work ~consumed\]
in symbols:
\[−Q_{c} = W\frac{T_{c}}{T_{h} − T_{c}}\]
\[Q_{h} = −Q_{c} + W\]
and in everyday language:
The closer the two temperatures are, the easier it is to move heat from cold to hot
where the temperatures are measured on an absolute scale. The hot object receives not only the heat removed from the cold object but also an amount of heat equal to the work consumed by the transfer. Note also that the work needed to remove heat from your home approaches infinity as its temperature approaches absolute zero; that’s why absolute zero is unattainable.
Sadly, a practical air conditioner never reaches ideal efficiency, so it moves less heat than promised by above equation. Moreover, heat leaks back into your home through its walls at a rate that’s roughly proportional to the temperature difference. No wonder your electric bill soars when you cool your home to arctic temperatures in tropical weather!
How an Air Conditioner Cools the Indoor Air
Having determined the air conditioner’s goals, we can now look at how a real air conditioner meets them. In most cases, the air conditioner uses a fluid to transfer heat from the colder indoor air to the hotter outdoor air. Known as the working fluid, this substance absorbs heat from the indoor air and releases that heat to the outdoor air. The working fluid flows in a looping path through the air conditioner’s three main components: an evaporator, a condenser, and a compressor. The evaporator is located indoors, where it transfers heat from the indoor air to the working fluid. The condenser is located outdoors, where it transfers heat from the working fluid to the outdoor air. The compressor is also located outdoors, where it squeezes the working fluid and does the work needed to move heat against its natural flow. To see how these three components pump heat out of your home, let’s look at them individually.
We’ll begin with the evaporator, a long metal pipe that’s decorated with thin metal fins. The evaporator is a heat exchanger that allows heat to flow from the warm indoor air around it to the cool working fluid inside it. Its fins provide additional surface area through which heat can flow, and a fan blows indoor air rapidly past those fins to encourage that flow. True to its name, the evaporator is where the working fluid evaporates from liquid to gas. To evaporate, the liquid working fluid needs its latent heat of evaporation, the energy required to separate a liquid’s molecules so that it becomes a gas. The working fluid obtains part of that energy from its own thermal energy, and its temperature decreases. Heat from the indoor air then flows into the chilled working fluid and completes the evaporation. By the time the working fluid leaves the evaporator as a gas, it has absorbed a great deal of the indoor air’s thermal energy and carries that energy with it as chemical potential energy.
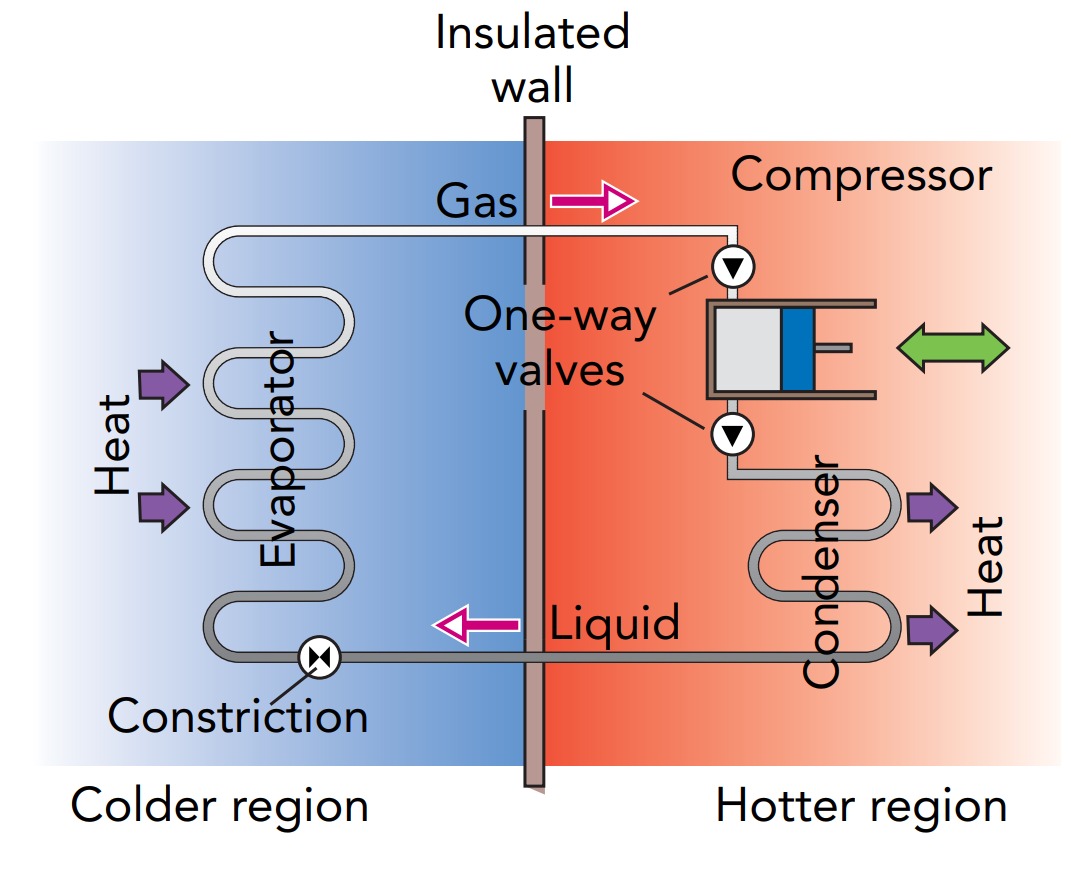
To make the working fluid evaporate, the air conditioner abruptly reduces its pressure. The phase transitions such as evaporation depend on molecular landing and leaving rates, and that those rates are sensitive to pressure and temperature. Working fluid flows toward the evaporator as a warm, high-pressure liquid, but its pressure drops dramatically when it flows into the evaporator through a narrow constriction in the tubing. That pressure drop slows the rate at which working fluid molecules land on the liquid from the gas so that molecules leaving the liquid for the gas dominate and the liquid starts to evaporate.
As the working fluid evaporates, its temperature plummets and its evaporation slows down; molecules rarely gather enough thermal energy from the cold liquid to leave it for the gas. However, heat from the warmer room air now rushes into the cold working fluid and encourages further evaporation. By the time the working fluid emerges from the evaporator, it has evaporated completely and has absorbed considerable thermal energy from the indoor air. It leaves the evaporator as a cool low-pressure gas and travels through a pipe toward the compressor. Half the air conditioner’s job is done: it has removed heat from the indoor air. The remaining half of its job is more challenging; it must add heat to the outdoor air while ensuring that the total entropy of the combined system doesn’t decrease. After all, there’s no getting around the law of entropy.
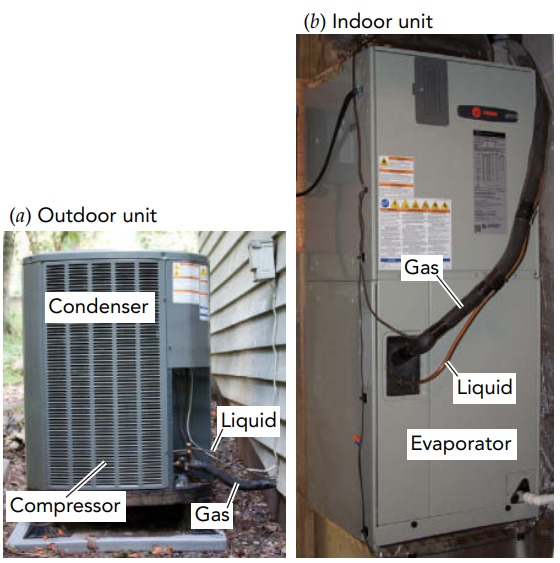
How an Air Conditioner Warms the Outdoor Air
Satisfying the law of entropy is the task of the compressor, an electrically powered device that squeezes gas into a smaller volume. The compressor receives low-pressure gaseous working fluid from the evaporator, compresses it to much higher density, and delivers it as a high-pressure gas to the condenser. The compressor may use a piston and one-way valves, like the water pump, or it may use a rotary pumping mechanism. Regardless of how it functions, the result is the same: the gaseous working fluid undergoes a dramatic increase in density and pressure as it passes through the compressor.
The compressor does work on the gas while compressing it—pushing the gas inward as the gas moves inward. In accordance with the law of conservation of energy, this work increases the internal energy of the working fluid. The only way that a gas can store additional energy is as thermal energy, so the working fluid leaves the compressor much hotter than when it arrived. There is no getting around that temperature rise; compressing a gas unavoidably raises its temperature.
Hot, high-pressure working fluid then flows into the condenser. Like the evaporator, the condenser is a long metal pipe with fins attached to it. It acts as a heat exchanger, and its fins provide extra surface area to speed the flow of heat from the hotter working fluid inside it to the less hot outdoor air. There may also be a fan to move outdoor air quickly past the condenser and speed up the heat transfer.
As its name suggests, the condenser is where the working fluid condenses from gas to liquid. That condensation starts when the gas begins to cool after compression. While it flows through a pipe toward the compressor, the low-pressure working fluid is stable as a gas. But in the high-pressure, high-density working fluid that emerges from the compressor and starts to cool in the condenser, the landing rate outpaces the leaving rate and the gas begins to condense. As its molecules bind together to form a liquid, the hot gaseous working fluid releases its latent heat of evaporation. This chemical potential energy becomes thermal energy in the working fluid, keeping it hot so that heat continues to flow out of it through the walls of the condenser to the cooler outdoor air.
By the time the working fluid leaves the condenser as a liquid, it has transformed a great deal of chemical potential energy into thermal energy and released that energy into the outdoor air. The outdoor air receives as heat not only the thermal energy extracted from the indoor air but also the electric energy consumed by the compressor. The working fluid exits the condenser as a warm, high-pressure liquid and travels through a pipe toward the evaporator.
The second half of the air conditioner’s job is now complete; it has released heat into the outdoor air and, in the process, converted ordered energy into thermal energy. From here, the working fluid returns to the evaporator to begin the cycle all over again. The working fluid passes endlessly around the loop, extracting heat from the indoor air in the evaporator and releasing it to the outdoor air in the condenser. The compressor drives the whole process and thereby satisfies the law of entropy.
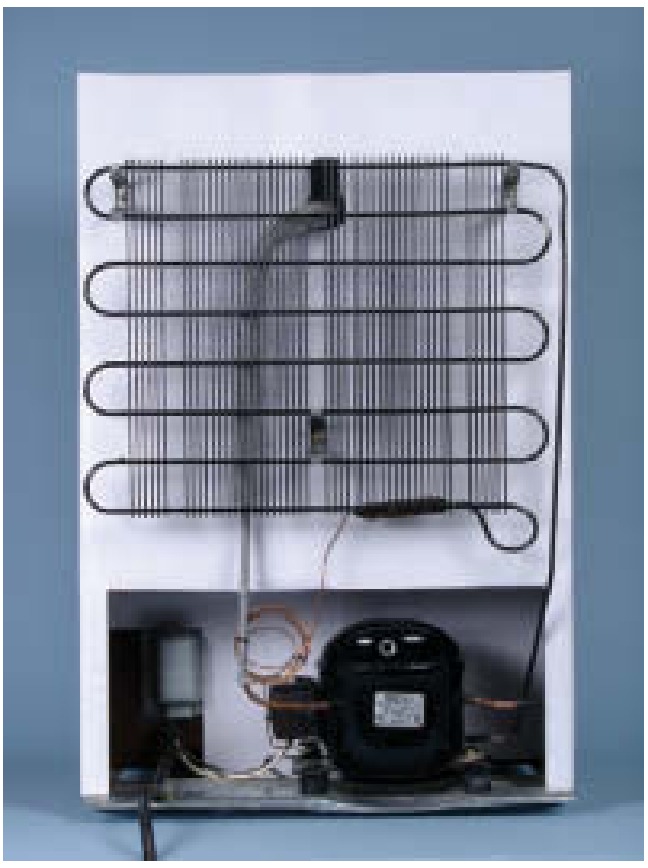
In fact, heat pumps of this type also appear in many common appliances. A refrigerator uses a heat pump to extract heat from food and release that heat to the room air. A drinking fountain uses a heat pump to transfer heat from water to the room air. Like all heat pumps, these cooling devices release more heat to the warm object (room air) than they extract from the cold object (food or water). That’s why when you hold the refrigerator door open and it begins to pump heat from one portion of room air to another portion of room air, the room gets warmer overall—the electric energy the refrigerator consumes while operating is being turned wastefully into thermal energy in the room. To save energy, keep the refrigerator closed whenever possible.
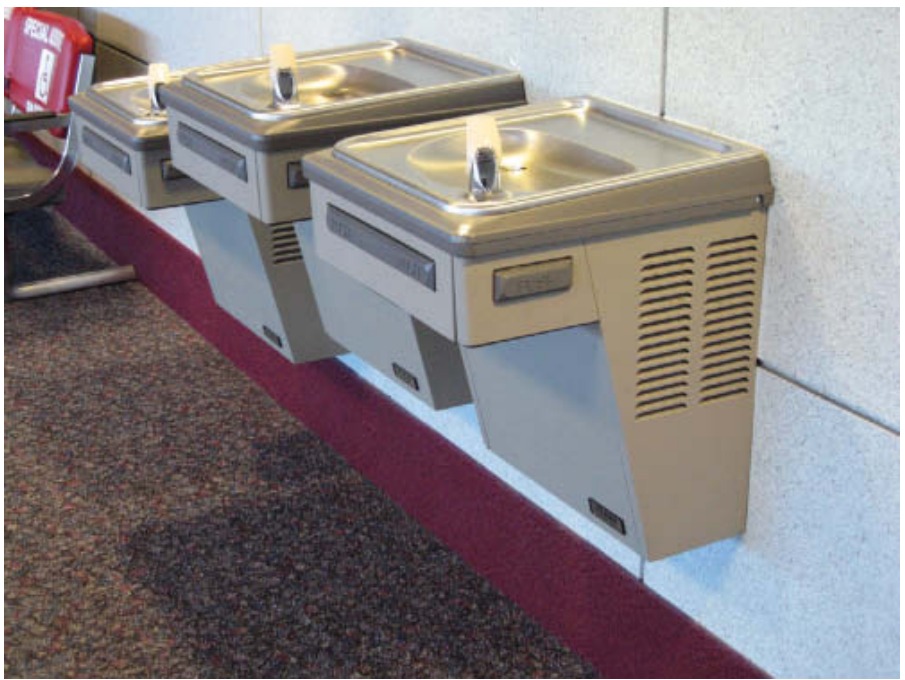
Many homes in moderate climates are heated by effectively running their air conditioners backward. Called heat pumps rather than air conditioners, these systems are capable of pumping heat against its natural direction of flow in winter as well is in summer.
In summer, a heat pump moves heat from indoor air to outdoor air to cool the home. In winter, however, it moves heat from outdoor air to indoor air to heat the home. Rather than turning electricity directly into thermal energy to heat the home, it leverages that electrical energy by using it to gather the abundant thermal energy from outdoors and to carry that indoors. Before leaving air conditioners, we should take a moment to look at the working fluid itself. This fluid must become a gas at low pressure and a liquid at high pressure, over most of the temperature range encountered by the air conditioner. For decades, the standard working fluids were chlorofluorocarbons such as the various Freons. These compounds replaced ammonia, a toxic and corrosive gas used in early refrigeration.
Chlorofluorocarbons are ideally suited to air conditioners because they easily transform from gas to liquid and back again over a broad range of temperatures. They’re also chemically inert and inexpensive. Unfortunately, chlorofluorocarbon molecules contain chlorine atoms and, when released into the air, can carry those chlorine atoms to the upper atmosphere. There they promote the destruction of ozone molecules, essential atmospheric constituents that absorb portions of the sun’s ultraviolet radiation. Recently, chlorine-free hydrofluorocarbons have replaced chlorofluorocarbons as the working fluids in most air conditioners. Though not as energy efficient and chemically inert as the materials they replace, hydrofluorocarbons do not damage the ozone layer. They are, however, potent greenhouse gases and should not be released to the atmosphere.