Automobiles
Nothing is more symbolic of freedom and personal independence than an automobile. With its keys in your hand, you can go almost anywhere at a moment’s notice. The mechanism that makes this instant transportation possible is the internal combustion engine. Though it has been refined over the years, this engine’s basic design has changed little since it was invented more than a century ago. It uses thermal energy released by burning fuel to do the work needed to propel the car forward. That thermal energy can do work at all is one of the marvels of thermal physics.
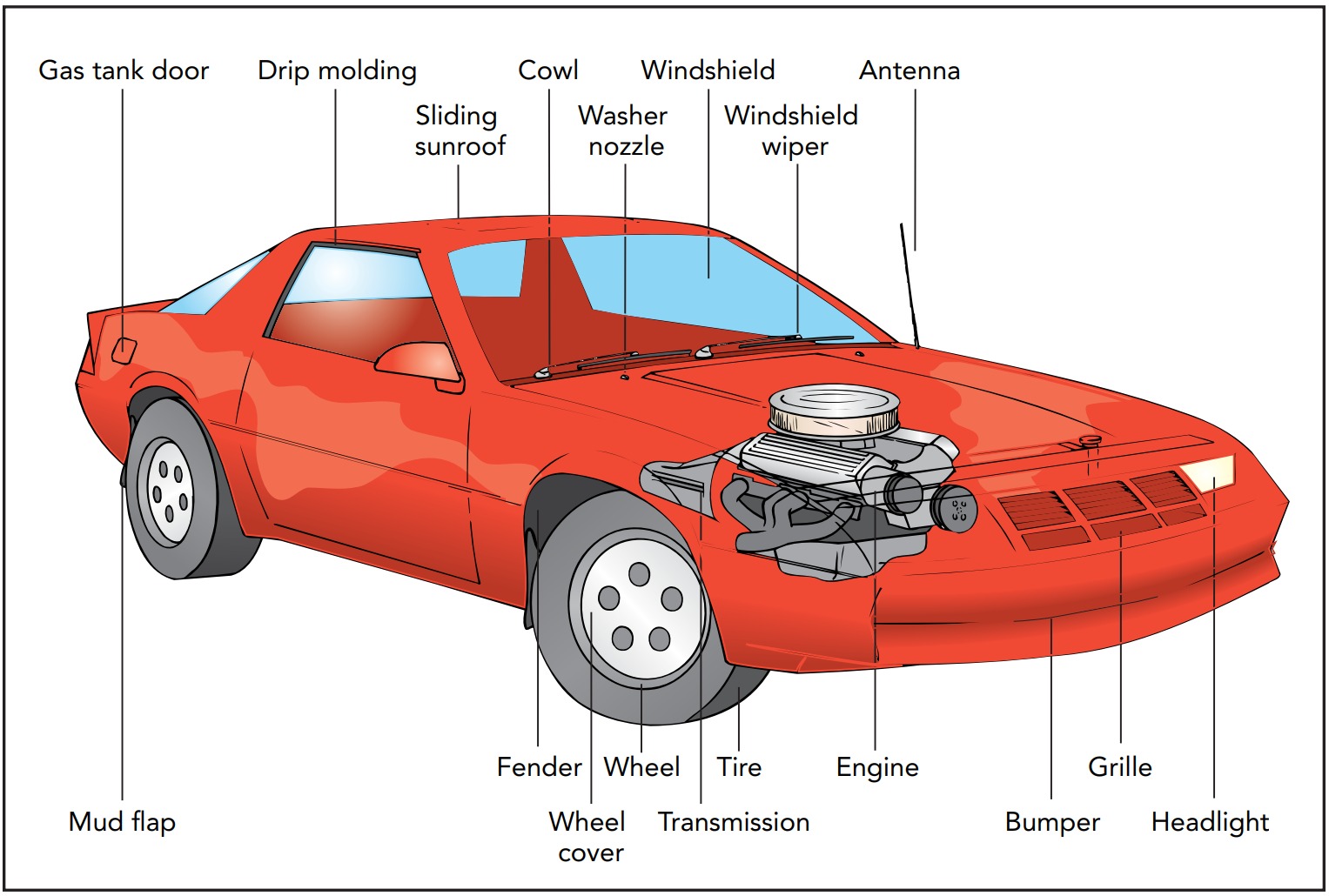
Using Thermal Energy: Heat Engines
The light turns green, and you step on the accelerator pedal. The engine of your car roars into action, and in a moment, you’re cruising down the road a mile a minute. The engine noise gradually diminishes to a soft purr and vanishes beneath the sound of the radio and the passing wind.
The engine is the heart of the automobile, pushing the car forward at the light and keeping it moving against the forces of gravity, friction, and air resistance. It’s not simply a miracle of engineering. It’s also a wonder of thermal physics because it performs the seemingly impossible task of converting thermal energy into ordered energy. However, the law of entropy forbids the direct conversion of thermal energy into ordered energy, so how can a car engine use burning fuel to propel the car forward?
The car engine avoids conflict with the law of entropy by being a heat engine, a device that converts thermal energy into ordered energy as heat flows from a hot object to a cold object. Although thermal energy in a single object can’t be converted into work, that restriction doesn’t apply to a system of two objects at different temperatures. Because heat flowing from the hot object to the cold object increases the overall entropy of the system, a small amount of thermal energy can be converted into work without decreasing the system’s overall entropy and without violating the law of entropy.
Another way to look at a heat engine is through the contributions of the two objects. The hot object provides the thermal energy that’s converted into work. The cold object provides the order needed to carry out that conversion. As the heat engine operates, the hot object loses some of its thermal energy and the cold object loses some of its order. The heat engine has used them to produce ordered energy. Since the heat engine needs both thermal energy and order, it can’t operate if either the hot or the cold object is missing. In a car engine, the hot object is burning fuel and the cold object is outdoor air. Some of the heat passing from the burning fuel to the outdoor air is diverted and becomes the ordered energy that propels the car. But what limits the amount of thermal energy the engine can convert into ordered energy?
To answer that question, let’s examine a simplified car engine. We’ll treat the burning fuel and outdoor air as a single, thermally isolated system and look at what happens to their total entropy as the engine operates. In accordance with the law of entropy, this total entropy can’t decrease while the engine is transforming some thermal energy into ordered energy
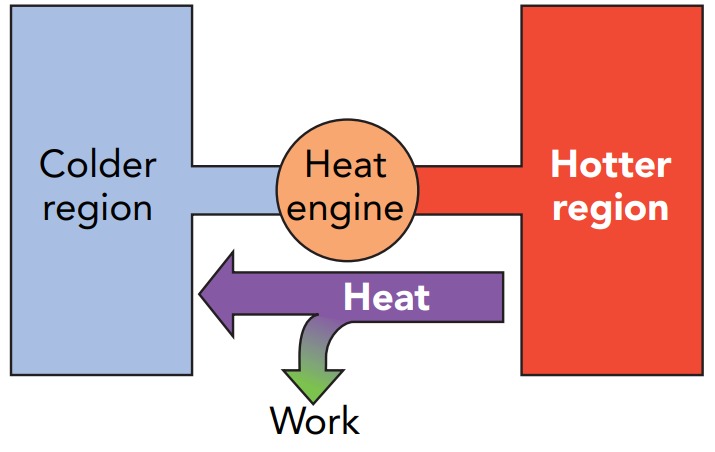
When the car is idling at a stoplight, its engine is doing no work and heat is simply flowing from the hot burning fuel to the cold outdoor air. The system’s total entropy increases because this heat is more disordering to the cold air it enters than to the hot burning fuel it leaves. In fact, the system’s entropy increases dramatically because the burning fuel is extremely hot compared to the cold outdoor air.
This increase in the system’s entropy is unnecessary and wasteful. The law of entropy requires only that the engine add as much entropy to the cold outdoor air as it removes from the hot burning fuel. Since a little heat is quite disordering to cold air, the car engine can deliver much less heat to the outdoor air than it removes from the burning fuel and still not cause the system’s total entropy to decrease. As long as the engine delivers enough heat to the outdoor air to keep the total entropy from decreasing, there’s nothing to prevent it from converting the remaining heat into ordered energy!
This conversion starts as soon as you remove your foot from the brake and begin to accelerate forward. Instead of transferring all the thermal energy in the burning fuel to the outdoor air, your car then extracts some of it as ordered energy and uses it to power the wheels. The car engine can convert thermal energy into ordered energy, as long as it passes along enough heat from the hot object to the cold object to satisfy the law of entropy. Obeying the law of entropy becomes easier as the temperature difference between the two objects increases. When the temperature difference is huge, as it is in an automobile engine, a large fraction of the thermal energy leaving the hot object can be converted into ordered energy—at least in theory.
For an ideally efficient automobile engine or other heat engine, the relationships among the heat removed from the hot object, the heat added to the cold object, and the work provided can be written as two word equations:
\[work ~provided = heat~ removed~ from ~ hot~ object ~ \frac{temperature ~hot − temperature_{cold}}{temperature_{hot}}\]
\[heat~ added~ to ~cold ~object = heat~ removed~ from ~hot~ object − work~ provided\]
in symbols:
\[W = −Q_{h}\frac{T_{h} − T_{c}}{T_{h}}\]
\[Q_{c} = −Q_{h} − W\]
and in everyday language:
The greater the temperature difference between hot and cold, the larger the fraction of heat you can divert and transform into work
where the temperatures are measured on an absolute scale. Unfortunately, theoretical limits are often hard to realize in actual machines, and the best automobile engines extract only about half the ordered energy specified by Eqs. Still, obtaining even that amount is a remarkable feat and a tribute to scientists and engineers who, in recent years, have labored to make automobile engines as energy efficient as possible.
The Internal Combustion Engine
Invented by the German engineer Nikolaus August Otto in 1867, the internal combustion engine burns fuel directly in the engine itself. Gasoline and air are mixed and ignited in an enclosed chamber. The resulting temperature rise increases the pressure of the gas and allows it to perform work on a movable surface.
To extract work from the fuel, the internal combustion engine must perform four tasks in sequence:
- It must introduce a fuel-air mixture into an enclosed volume.
- It must ignite that mixture.
- It must allow the hot burned gas to do work on the car.
- It must get rid of the exhaust gas.
In the standard, four-stroke fuel-injected engine found in modern gasoline automobiles, this sequence of events takes place inside a hollow cylinder. It’s called a fourstroke engine because it operates in four distinct steps, or strokes: induction, compression, power, and exhaust. Fuel-injected refers to the technique used to mix the fuel and air as they’re introduced into the cylinder.
Automobile engines usually have four or more of these cylinders. Each cylinder is a separate energy source, closed at one end and equipped with a movable piston, several valves, a fuel injector, and a spark plug. The piston slides up and down in the cylinder, shrinking or enlarging the cavity inside. The valves, located at the closed end of the cylinder, open to introduce fuel and air into the cavity or to permit burned exhaust gas to escape from the cavity. The fuel injector adds fuel to the air as it enters the cylinder. The spark plug, also located at the closed end of the cylinder, ignites the fuel-air mixture to release its chemical potential energy as thermal energy.
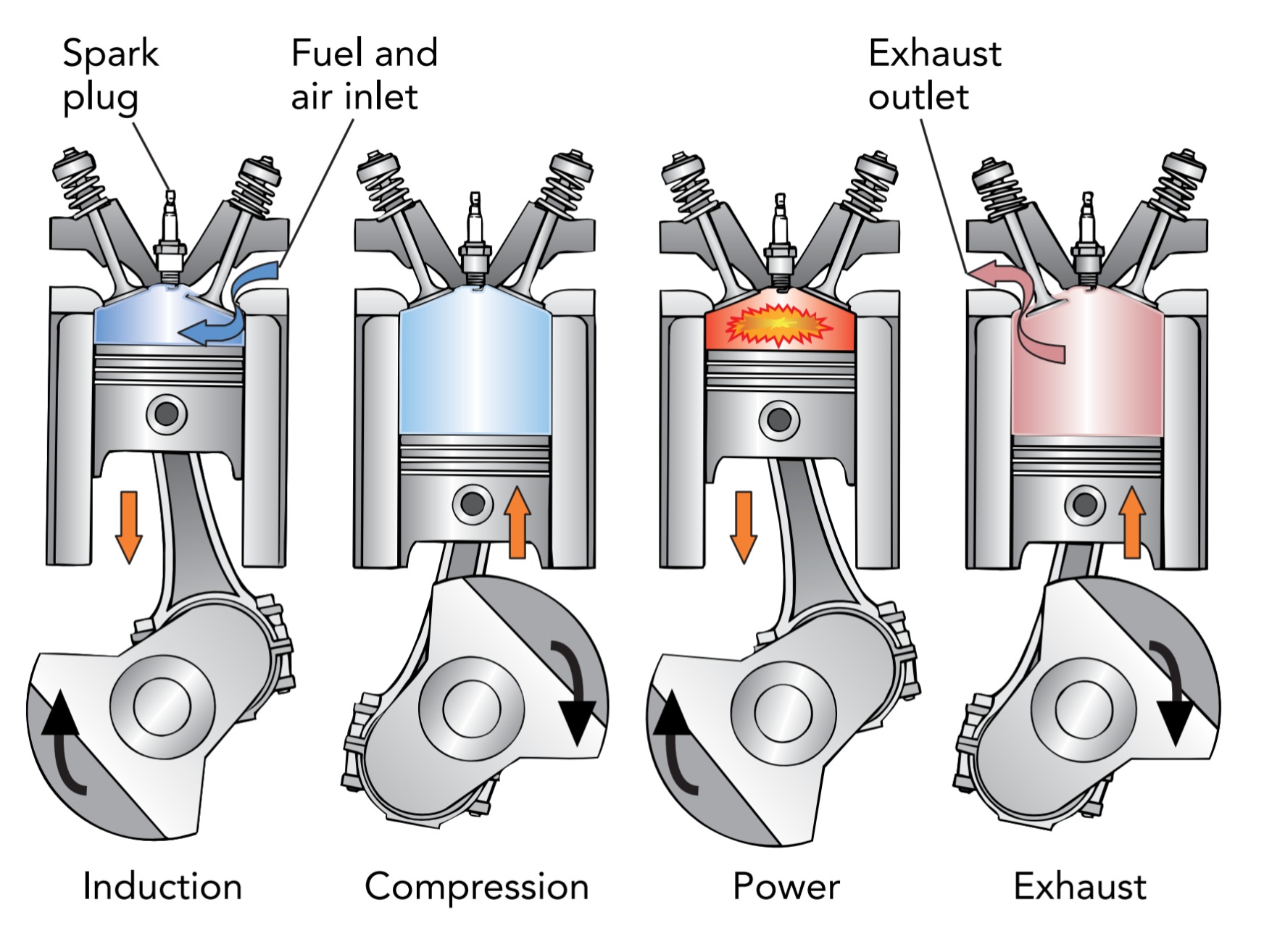
The fuel-air mixture is introduced into each cylinder during its induction stroke. In this stroke, the engine pulls the piston away from the cylinder’s closed end so that its cavity expands to create a partial vacuum. At the same time, the cylinder’s inlet valves open so that atmospheric pressure can push fresh air into the cylinder. The cylinder’s fuel injector adds a mist of fuel droplets to this air so that the cylinder fills with a flammable fuel-air mixture. Because it takes work to move air out of the way and create a partial vacuum, the engine does work on the cylinder during the induction stroke.
At the end of the induction stroke, the inlet valves close to prevent the fuel-air mixture from flowing back out of the cylinder. Now the compression stroke begins. The engine pushes the piston toward the cylinder’s closed end so that its cavity shrinks and the fuel-air mixture becomes denser. The engine does work on the mixture while compressing it—the piston pushes the gas inward as the gas moves inward—so the mixture’s internal energy increases. The only way that a gas can store this additional energy is as thermal energy, so the mixture’s temperature rises as it’s compressed. Since increases in a gas’s density and temperature both increase its pressure, the pressure in the cylinder rises rapidly as the piston approaches the spark plug.
At the end of the compression stroke, the engine applies a high-voltage pulse to the spark plug and ignites the fuel-air mixture. The mixture burns quickly to produce hot, high-pressure burned gas, which then does work on the car during the cylinder’s power stroke. In that stroke, the gas pushes the piston away from the cylinder’s closed end so that its cavity expands and the burned gas becomes less dense. Since the hot gas exerts a huge pressure force on the piston as it moves outward, it does work on the piston and ultimately propels the car. As it does work, the burned gas gives up thermal energy and cools in accordance with the law of conservation of energy. Its density and pressure also decrease. At the end of the power stroke, the exhaust gas has cooled significantly and its pressure is only a few times atmospheric pressure. The cylinder has extracted much of the fuel’s chemical energy as work.
The cylinder gets rid of the exhaust gas during its exhaust stroke. In this stroke, the engine pushes the piston toward the closed end of the cylinder while the cylinder’s outlet valves are open. Because the burned gas trapped inside the cylinder at the end of the power stroke is well above atmospheric pressure, it accelerates out of the cylinder the moment the outlet valves open. These sudden bursts of gas leaving the cylinders create the “poof-poofpoof” sound of a running engine. Without a muffler on its exhaust pipes, the engine would be loud and unpleasant.
Just opening the outlet valves releases most of the exhaust gas, but the rest is squeezed out as the piston moves toward the cylinder’s closed end. The engine again does work on the cylinder as it squeezes out the exhaust gas. At the end of the exhaust stroke, the cylinder is empty and the outlet valves close. The cylinder is ready to begin a new induction stroke.
Engine Efficiency
The goal of an internal combustion engine is to extract as much work as possible from a given amount of fuel. In principle, the fuel’s chemical potential energy can be converted entirely into work because both are ordered energies. However, it’s difficult to convert
chemical potential energy directly into work, so the engine burns the fuel instead. This step is unfortunate, for in burning the fuel, the engine converts the fuel’s chemical potential energy directly into thermal energy and produces lots of unnecessary entropy. But all is not lost. Since the burned fuel is extremely hot, a good fraction of its thermal energy can be converted into ordered energy by diverting some of the heat that flows from the burned fuel to the outdoor air. As we noted earlier, the hotter the burned fuel and the colder the outdoor air, the more ordered energy the engine can extract. To maximize its fuel efficiency, an internal combustion engine obtains the hottest possible burned gas, lets that gas do as much work as it can on the piston, and releases the gas at the coldest possible temperature.
It would be wonderful if, during the power stroke, the burned gas expanded and cooled until it reached the temperature of the outdoor air. The exhaust gas would then leave the engine with the same amount of thermal energy it had when it arrived, and the engine would have extracted all of the fuel’s chemical potential energy as work. Unfortunately, that would violate the law of entropy by converting thermal energy completely into ordered energy. An operating heat engine always adds some heat to its cold object. In this case, the engine releases the burned gas before it cools to the temperature of the outdoor air. It has no choice; the engine’s exhaust must be hot!
An ordinary internal combustion engine, however, is even less fuel efficient than the law of entropy requires. It releases the burned gas while that gas is still well above atmospheric pressure and is therefore still capable of doing work on the piston. By allowing that gas to expand further before release, an improved engine could extract more work from it and thus be more fuel efficient. The Atkinson cycle engine is just such a device, with a power stroke that is longer than its compression stroke. However, even if the engine expanded the burned gas until it reached atmospheric pressure, the temperature of its exhaust would still remain somewhat above the temperature of the outdoor air. Invented in 1882, Atkinson cycle engines have become popular only recently as part of the effort to build more fuel-efficient vehicles.
Regardless of these improvements, real internal combustion engines always waste some energy and extract less work than the law of entropy allows. For example, a fraction of the heat leaks from the burned gas to the cylinder walls and is removed by the car’s cooling system. This wasted heat isn’t available to produce work. Similarly, sliding friction in the engine wastes mechanical energy and necessitates an oil-filled lubricating system. Overall, real internal combustion engines convert only about 20–30% of the fuel’s chemical potential energy into work.
Improving Engine Efficiency
To obtain the hottest possible burned gas, the compression stroke should squeeze the fuelair mixture into as small a volume as possible. The more tightly the piston compresses the mixture, the higher its density, pressure, and temperature will be before ignition and the hotter the burned gases will be after ignition. Since the efficiency of any heat engine increases as the temperature of its hot object increases and since the hot burned gas is the automobile engine’s “hot object,” its high temperature after ignition is good for efficiency.
Approximate Ignition Temperatures for the Three Standard Grades of Gasoline During Compression
Octane Number | Approximate Ignition Temperature |
---|---|
87 (regular) | 750 °C (1382 °F) |
90 (plus) | 800 °C (1472 °F) |
93 (premium) | 850 °C (1562 °F) |
The extent to which the cylinder’s volume decreases during the compression stroke is measured by its compression ratio, its volume at the start of the compression stroke divided by its volume at the end of the compression stroke. The larger this compression ratio, the hotter the burned gas and the more energy efficient the engine. While normal compression ratios are between 8:1 and 12:1, those in high-compression engines may be as much as 15:1.
Unfortunately, the compression ratio can’t be made arbitrarily large. If the engine compresses the fuel-air mixture too much, the flammable mixture will become so hot that it will ignite all by itself. This spontaneous ignition due to overcompression is called preignition or knocking. When an automobile knocks, the gasoline burns before the engine is ready to extract work from it and much of the energy is wasted. There are two ways to reduce knocking. First, you can mix the fuel and air more uniformly. In a nonuniform mixture, there may be small regions of gas that get hotter or are more susceptible to ignition than others. The fuel-injection technique used in all modern cars provides excellent mixing and also allows a car’s computer to adjust the fuel-air mixture for complete combustion and minimal pollution. So unless a car is seriously out of tune, there isn’t much room for improvement as far as mixture uniformity is concerned.
Second, you can use the most appropriate fuel. Not all fuels ignite at the same temperature, so you should select a fuel that is able to tolerate your car’s compression process without igniting spontaneously. That’s exactly what you do when you purchase the proper grade of gasoline. Regular gasoline ignites at a relatively low temperature and is most susceptible to knocking. Premium gasoline ignites at a relatively high temperature and is most resistant to knocking.
Fuels that are more difficult to ignite and more resistant to knocking are assigned higher octane numbers. Regular gasoline has an octane number of about 87, while premium has an octane number of about 93. Choosing the proper fuel is simply a matter of finding the lowest octane gasoline that your car can use without excessive knocking. A little knocking in the most demanding circumstances is quite acceptable. Most modern well-tuned automobiles work beautifully on regular gasoline. Since only high-performance cars with high-compression engines need premium gasoline, putting anything other than regular gasoline in a normal car is usually a waste of money.
Diesel Engines and Turbochargers
Since knocking sets the limit for compression ratio, it also sets the limit for efficiency in a gasoline engine. However, diesel engines avoid the knocking problem by separating the fuel and air during the compression stroke. Invented by German engineer Rudolph Christian Karl Diesel (1858–1913) in 1896, the diesel engine has no spark plug to ignite the fuel. Instead, it compresses pure air with an extremely high compression ratio of perhaps 20:1 and then injects diesel fuel directly into the cylinder just as the power stroke begins. The fuel ignites spontaneously as it enters the hot compressed air. Unlike an internal combustion engine, which uses a fuel that is difficult to ignite, a diesel engine uses fuel that ignites easily.
Because of its higher compression ratio, a diesel engine burns its fuel at a higher temperature than a standard gasoline engine and can therefore be more energy efficient. It effectively has a hotter “hot object” and can convert a larger fraction of heat into work. Some gasoline or diesel engines combine fuel injection with a turbocharger. A turbocharger is essentially a fan that pumps outdoor air into the cylinder during the induction stroke. By squeezing more fuel-air mixture into the cylinder, a turbocharger increases the engine’s power output. The engine burns more fuel with each power stroke and behaves like a larger engine.
The fan of a turbocharger is powered by pressure in the engine’s exhaust system. A nearly identical device called a supercharger is driven directly by the engine’s output power. For an internal combustion engine, turbochargers have a downside: they encourage knocking. As a turbocharger squeezes air into the cylinder, it does work on that air and the air becomes hot. Since the fuel-air mixture enters the engine hot, it may ignite spontaneously during the compression stroke. To avoid knocking in a car equipped with a turbocharger, you may need to use premium gasoline. Some turbocharged cars are equipped with an intercooler, a device that removes heat from the air passing through the turbocharger. By providing cool, high-density air to the cylinders, the intercooler reduces the peak temperature of the compression stroke and avoids knocking.
For diesel engines, however, knocking is not an issue. In fact, turbochargers have revolutionized diesel engines, transforming them from sluggish to high performance. Because of their high compression ratios and extremely hot combustion temperatures, diesel engines develop enormous pressures inside their cylinders and tremendous forces on their pistons. As a result, diesel engines can provide large torques and enormous powers, even at low engine speeds.
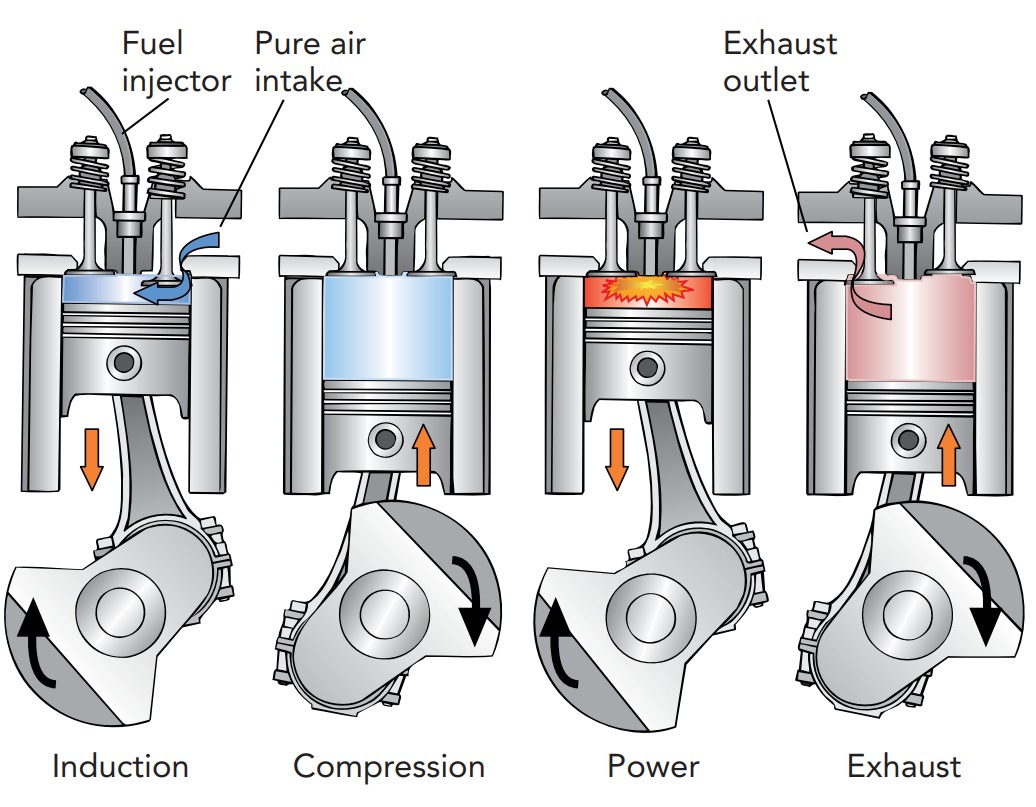
With higher fuel efficiency and enhanced performance, these modern diesel engines have a clear environmental advantage over gasoline engines. However, they still had to solve a long-standing problem with diesel engines—particulate pollution. Because a diesel engine must burn its oily liquid fuel in about 1/1000th of a second, it was difficult to burn the fuel completely and some of it became carbon soot. To eliminate that soot, diesel engines were reengineered to swirl their fuel and air vigorously for more complete burning and they now incorporate filters that capture and burn up any soot that does manage to get into the exhaust system. The result is a relatively fuel-efficient vehicle with environmental specifications that rival hybrid vehicles.
Multicylinder Engines
Since the purpose of the engine is to extract work from the fuel-air mixture, it’s important that each cylinder do more work than it consumes. Three of the strokes require the engine to do work on various gases, and only one of the strokes extracts work from the burned gas. During the induction stroke, the engine does work drawing the fuel-air mixture into the cylinder. During the compression stroke, the engine does work compressing the fuel-air mixture. During the exhaust stroke, the engine does work squeezing the exhaust gas out of the cylinder. Fortunately, the work done on the engine by the hot burned gas during the power stroke more than makes up for the work the engine does during the other three strokes. Still, the engine has to invest a great deal of energy into the cylinder before each power stroke. To provide this initial energy, most four-stroke engines have four or more cylinders, timed so that there is always one cylinder going through the power stroke. The cylinder that is in the power stroke provides the work needed to carry the other cylinders through the three nonpower strokes, and there is plenty of work left over to propel the car itself.
While the pistons move back and forth, the engine needs a rotary motion to turn the car’s wheels. The engine converts each piston’s reciprocating motion into rotary motion by coupling that piston to a crankshaft with a connecting rod. The crankshaft is a thick steel bar, suspended in bearings, that has a series of pedal-like extensions, one for each cylinder. As the piston moves out of the cylinder during the power stroke, it pushes on the connecting rod and the connecting rod pushes on its crankshaft pedal. The connecting rod thus produces a torque on the crankshaft. The crankshaft rotates in its bearings and transmits this torque out of the engine so that it can be used to propel the car. So, while each cylinder initially exerts a force, the crankshaft uses that force to produce a torque.
The spinning crankshaft conveys its rotary power to the car’s transmission, and from there the power moves on to the wheels. Overall, a significant portion of the heat flowing out of the burning fuel-air mixture is being converted into work and used to spin the car’s wheels. Assisted by friction with the pavement, the wheels push the car forward, and you cruise down the highway toward your destination.