Physics of Nucleus
Although our access to most forms of high-energy radiation is restricted, there's one source that anyone can use—the sun. Because the sun's ultraviolet light is energetic enough to damage chemical bonds and rearrange molecules, it can provide us with a glimpse of the effects that occur with X-rays and beyond.
In recent years, scientists have been looking deeper into the atom to see how it's made, farther into space to see how the universe works, and more carefully into matter and motion to see how complicated things can be understood in terms of simple laws. Among the most important tools that these scientists have to work with are quantum theory and the theory of relativity.
To see sun damage for yourself, expose some sheets of colored construction paper to direct sunlight for a few days. Cover the paper with some opaque objects such as coins and place it outdoors. Don't cover the paper with glass because glass absorbs enough ultraviolet light to slow the damage process. After a day or two, you should find that the exposed portions of the paper have lightened; the sun's ultraviolet radiation has destroyed some of the dye molecules in the paper. This is known as optical bleaching. If you find that nothing happens, the dye is evidently robust enough to tolerate ultraviolet light for a while.
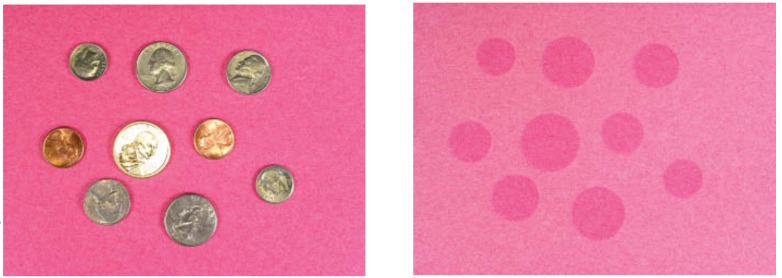
The optical bleaching appears on items that are displayed in shop windows and on outdoor furniture. It was once the only method people had for whitening fabrics. The sun's ultraviolet light also damages your skin when you sit in the sun - a sun burn isn't thermal damage; it's radiation damage. Fortunately ultraviolet light can't penetrate far into your body. In this unit, we will be considering the damage caused by a radiation, especially, nuclear energy.
Nuclear Weapons
The atomic bomb is one of the most remarkable and infamous inventions of the twentieth century. It followed close on the heels of various developments in the understanding of nature, developments that in many ways made the invention of nuclear weapons inevitable. By the late 1930s, scientists had discovered most of the principles behind nuclear energy and were well aware of how those principles might be applied. The onset of World War II prompted concern that Germany would choose to follow the military path of nuclear energy. Propelled by fear, curiosity, and temptation, the scientists, engineers, and politicians of that time brought nuclear weapons into existence. The world has lived in the shadow of these terrible devices ever since.
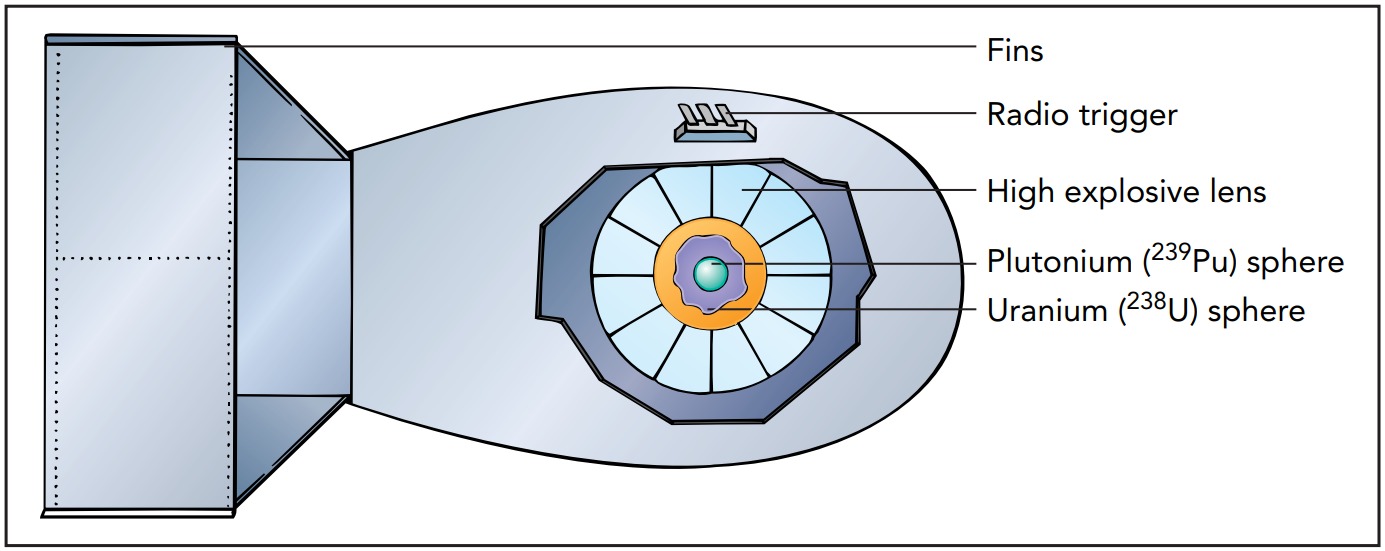
At the end of the nineteenth century, classical physics reigned supreme. Here classical physics means the rules of motion and gravitation identified by such people as Galileo, Newton, and Kepler, and the rules of electricity and magnetism developed by others, including Ampère, Coulomb, Faraday, and Maxwell. It was generally felt that most of physics was well understood. Physicists knew all the laws governing the behavior of objects in our universe, and all that was left to do was to apply those laws to more and more complicated examples. It was a time when physicists didn't know what they didn't know.
However, a few nagging problems remained—specific difficulties that couldn't be explained by the rules of classical physics. Among these were the spectrum of light emitted by a blackbody, the photoelectric effect in which electrons are ejected from metals by light, and the apparent absence of an ether or medium in which light traveled. At the beginning of the twentieth century, the whole of classical physics collapsed under the weight of these seemingly trivial difficulties, and a largely new understanding of the universe emerged. The major advances took 25 years, from 1901 to 1926, and the time since has largely been spent applying those new laws to more and more complicated examples.
The two main developments, both essential to the making of the atomic bomb, were the discoveries of quantum physics and relativity. Often these are called quantum theory and the theory of relativity. But while the word theory might imply that they're somehow on shaky ground, they're not theories in the sense of hypotheses waiting to be tested. In fact, they've been confirmed countless times since they were developed and have been shown to have enormous predictive power. Rather, they're theories in the sense of being carefully constructed and codified rules that model the behavior of the physical universe in which we live. Between them, these two theories made the discovery of nuclear forces and nuclear energy unavoidable. Finally, given people's love for gadgets and power, they also made nuclear weapons inevitable.
The Nucleus and Radioactive Decay
Though the name atomic bomb has stuck for more than half a century, the more correct name would be nuclear bomb. The items that are responsible for the energy released by nuclear weapons are not atoms but tiny pieces of atoms - their nuclei.
Let's start by looking at atoms. To get an idea of just how tiny atoms are, imagine magnifying a grain of table salt, which is 1 mm on a side, until it is the size of the state of Colorado. That grain would then appear as an orderly arrangement of spherical particles, each about the size of a grapefruit. These spherical particles would be single atoms, and there would be about 7.2 million of them along each edge of the grain.
Like most solids, table salt is a crystal and its atoms are bound to one another by their outermost components, their electrons. Electrons dominate the chemistry of atoms and molecules. Sodium is a reactive metal because of its electrons, and chlorine is a reactive gas because of its electrons. When mixed, these two chemicals react violently to form table salt and release a considerable amount of light and heat. This, then, is a true atomic bomb.
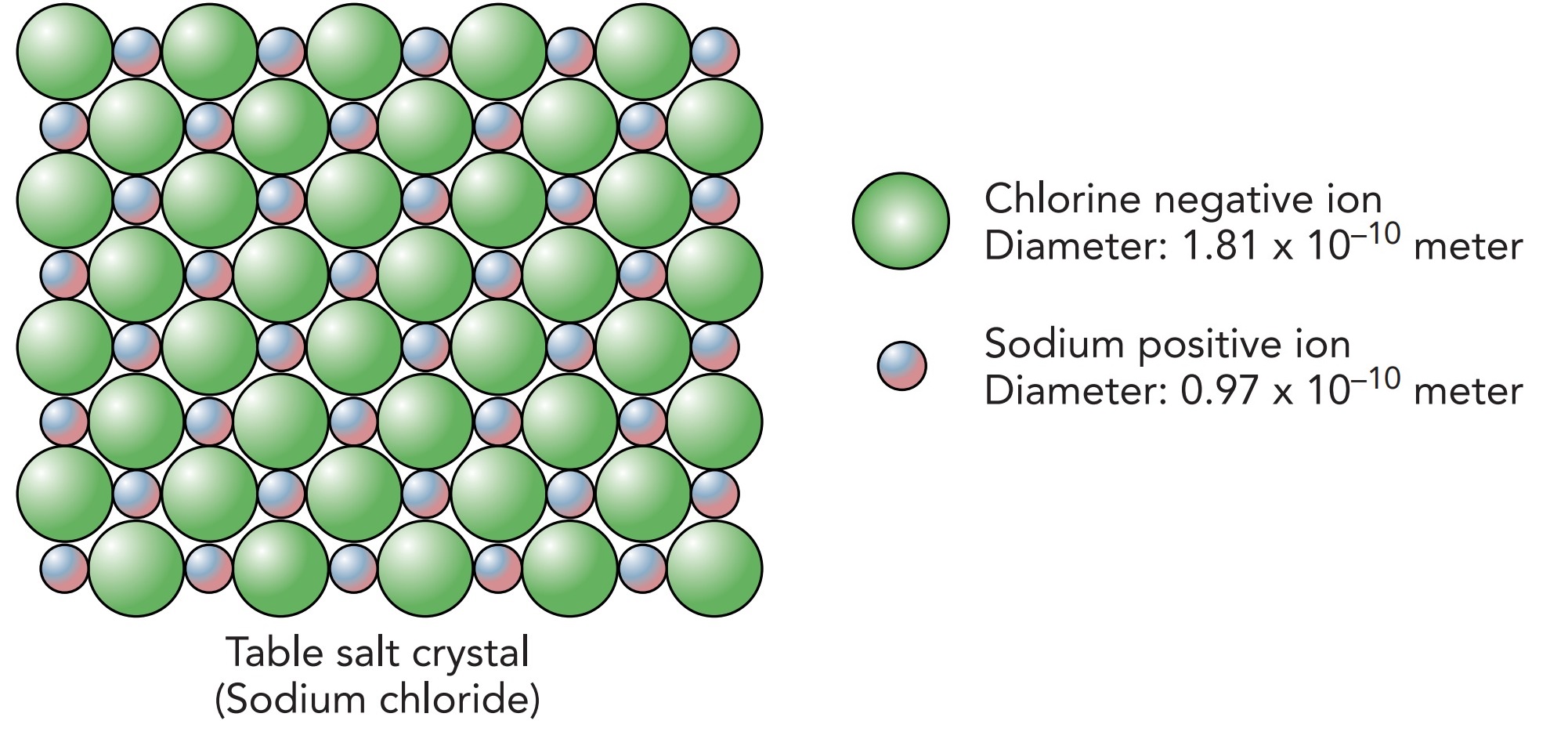
Obviously, something is missing here. If a crazy person could buy a kilogram or two of sodium and a tank of chlorine from a chemical company and destroy an entire city, rural living would be a whole lot more popular. Fortunately the energy released by chemical reactions is fairly limited. A kilogram of chemical atomic explosives just can't do that much damage. However, nuclear bombs tap an entirely different store of energy deep within the atoms.
Although all nuclear weaponry is often attributed to Einstein's famous equation, E = mc square,
that notion is vastly oversimplified. Nonetheless, this equation is quite significant. One of Einstein's discoveries at the beginning of the twentieth century
was that matter and energy are in some respects equivalent. In certain circumstances, mass can become energy or energy can become mass. This equivalence is part of the theory of
relativity and has some interesting consequences. It implies that an object can reduce its
mass by transferring energy to its surroundings. Thus, if you weigh an object before and
after it undergoes some internal transformation, you can use any weight loss to determine
how much energy was released from the object by that transformation.
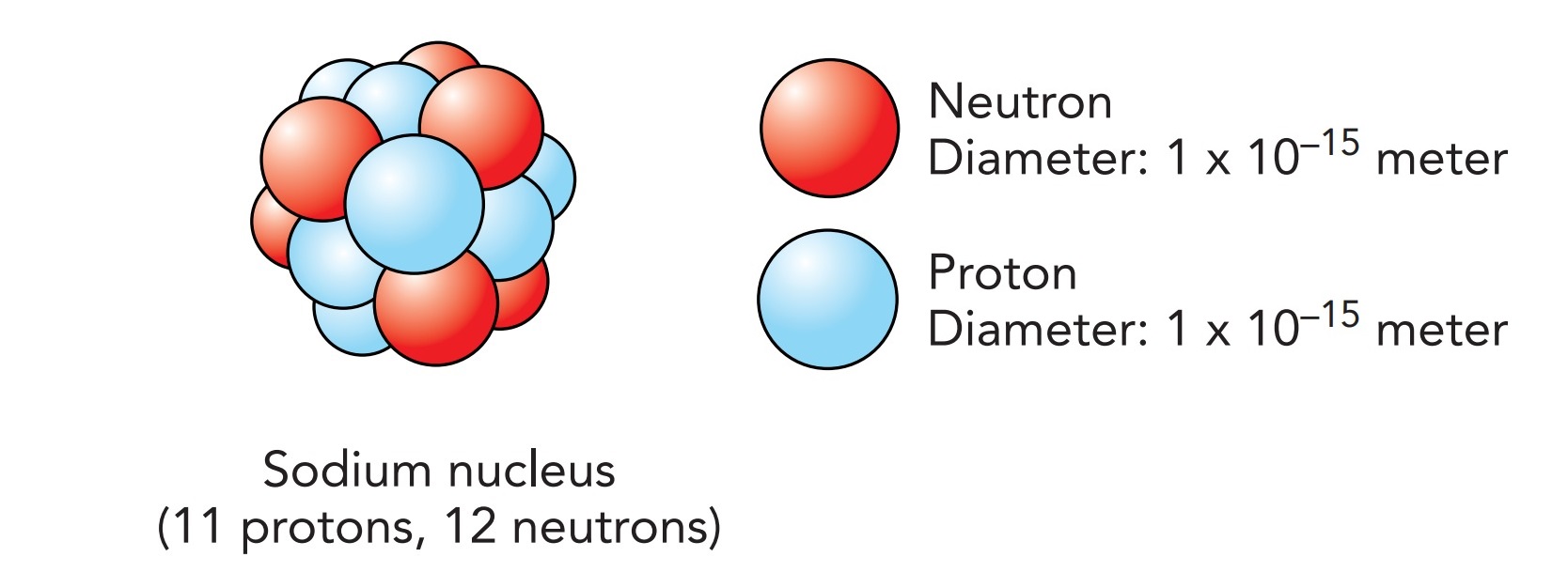
Because of this equivalence, mass and changes in mass can be used to locate energy that's hidden within normal matter. This technique is important in nuclear physics, but it also applies to chemistry. When sodium and chlorine react to form table salt, their combined mass decreases by a tiny amount. What's missing is some chemical potential energy, which becomes light and heat and escapes from the mixture. In leaving, this chemical potential energy reduces the mass of the sodium and chlorine mixture by about 1 part in 10 billion. That tiny change in mass is too small to detect with present measuring devices, although scientists are working on techniques that will soon make it possible to measure mass changes due to forming chemical bonds.
Electrons are, however, by far the lightest part of an atom and thus have relatively little mass to release as energy. Most of an atom's mass is located in its nucleus. The nucleus is fantastically small—only a little more than 10 minus 15 meter in diameter. If you were to peer into one of the grapefruit-size sodium ions of our giant salt crystal, you would see a tiny particle at its center. There, just at the threshold of visibility, would be the ion's nucleus, only 1 micrometer in diameter. The remaining 99.9999999999999% of the ion is occupied only by its 10 electrons in their orbitals.
The sodium nucleus contains 11 protons and 12 neutrons. Each of these nuclear particles, or nucleons, has about 2000 times as much mass as an electron, so 99.975% of the sodium ion's mass is in this nucleus. Thus, while the electrons are certainly important to chemistry and matter as we know it, their contribution to the ion's mass is insignificant. The ion is mostly empty space, lightly filled with fluffy electrons and having a tiny nuclear lump at its center.
The nucleons that make up this nucleus experience two competing forces. The first of these forces is the familiar electrostatic repulsion between like electric charges. Because each proton in the nucleus has a single positive charge, they're constantly trying to push one another out of the nucleus. However, the second of these forces is attractive and holds the nucleus together. This new force is called the nuclear force, and at short distances it dominates the weaker electrostatic repulsion. However, the nuclear force attracts the nucleons toward one another only when they're touching. As soon as they're separated, they're on their own.
The competition between these two forces—the repulsive electrostatic force between like charges and the attractive nuclear force between nucleons. The attractive nuclear force prevents the nucleus from coming apart, despite the enormous amount of electrostatic potential energy it contains. The nuclear force creates an energy barrier that prevents the nucleons from separating. Unless something adds energy to the nucleus to help the nucleons break free of the nuclear force, the nucleus will remain together forever. At least, that's the prediction of classical physics.
Quantum physics, however, has an important influence on the behavior of the nucleus. One of the many peculiar effects of quantum physics is that you can never really tell exactly where an object is located, or at least not for long. That fuzziness is a manifestation of the Heisenberg uncertainty principle, which observes that some pairs of physical quantities, such as position and momentum or energy and time, are not entirely independent and cannot be determined simultaneously beyond a certain accuracy. This principle is a result of the partly wave and partly particle nature of objects in our universe. Since waves are normally broad things that occupy a region of space rather than a single point, objects in our universe normally don't have exact locations.
The smaller an object's mass, the fuzzier it is and the more uncertain its location. Although the fuzzy nucleons in a nucleus will normally stay in contact with one another for an extremely long time, there's always a tiny chance that they'll find themselves temporarily separated by a distance that's beyond the reach of the nuclear force. The nucleons will then suddenly be free of one another, and electrostatic repulsion will push them apart in a process called radioactive decay. The quantum process that allows the nucleons to escape from the nuclear force without first obtaining the energy needed to surmount the energy barrier is called tunneling because the nucleons effectively tunnel through the barrier. We first encountered quantum tunneling.
Natural radioactive decay is a perfectly random process. Although half of a large population of identical radioactive nuclei will decay in a certain amount of time, you absolutely cannot predict in advance which of the original nuclei will have survived. Because of this randomness, radioactive decay is characterized simply by a half-life, the time required for half of the nuclei to decay. After one half-life, only half the original nuclei will remain intact. If you wait a second half-life, only a quarter of the original nuclei will remain (half of the remaining half). After a third half-life, only an eighth will remain (half of the remaining half of a half) and so on.
This halving of the population with each additional half-life is a type of exponential decay. In general, the fraction of nuclei remaining after a given amount of time is one-half raised to the power of the time divided by the half-life.
\[\frac{N}{N_0}=\left(\frac{1}{2}\right)^{\frac{t}{T_{\frac{1}{2}}}}\]
and in everyday language: Radioactivity goes away, but you have to wait it out
Although most radioactive nuclei have short half-lives and don't linger long in our environment, there are a few with half-lives of billions of years. It is those long-lived radioactive nuclei, particularly uranium and thorium nuclei, that have survived since the formation of Earth, remain abundant in nature, and gave rise to nuclear weapons.
Fission and Fusion
The more protons there are in a nucleus, the more they repel one another and the more likely they are to cause radioactive decay. Adding additional neutrons to the nucleus reduces this proton-proton repulsion by increasing the size of the nucleus without adding to its positive charge. However, adding too many neutrons also destabilizes the nucleus. Thus, constructing a stable nucleus is a delicate balancing act.
In nuclei with only a few protons, the attractive nuclear force wins big over the repulsive electrostatic force and the nucleons stick like crazy. These nuclei resemble hopping toys with weak springs and big suction cups; once brought together, the pieces never come apart. In fact, the average binding energy of the nucleons (the energy required to separate them from one another divided by the number of nucleons) would increase if these nuclei had even more protons and neutrons.
In nuclei with many protons, the electrostatic repulsion is so severe that the nuclear force can't hold the nucleons together for long. These nuclei decay rapidly. They resemble hopping toys with strong springs and small suction cups. The average binding energy of the nucleons would increase if these nuclei had fewer protons and neutrons.
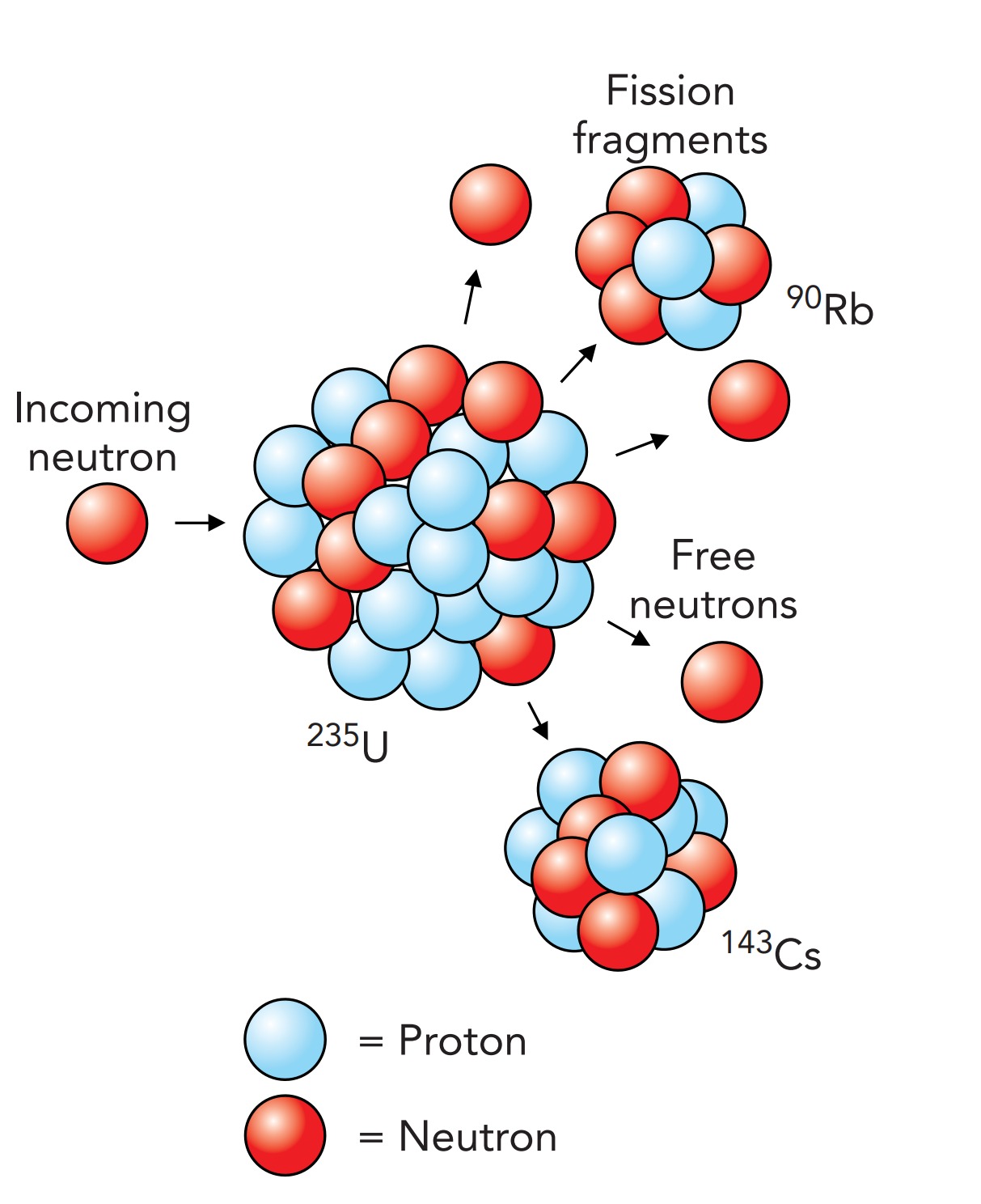
In nuclei with roughly 26 protons, in between the two extremes we've just considered, the attractive nuclear force and repulsive electrostatic force are nicely balanced. These nuclei are extremely stable, and you can't increase the average binding energy of their nucleons by adding or subtracting nucleons. Smaller nuclei can release potential energy by growing to reach this intermediate size, while larger nuclei can release potential energy by shrinking toward the same goal.
For a small nucleus to grow, something must push more nucleons toward it. Electrostatic repulsion will initially oppose this growth, but once everything touches, the nuclear force will bind the particles together and release a large amount of potential energy. This coalescence process is called nuclear fusion.
For a large nucleus to shrink, something must separate its pieces beyond the reach of the nuclear force. Electrostatic repulsion will then push the fragments apart and release a large amount of potential energy. This fragmentation process is called nuclear fission.
The energies released when small nuclei undergo fusion or when large nuclei undergo fission are enormous compared to chemical energies. Uranium, a large nucleus, converts about 0.1% of its mass into energy when it breaks apart. Hydrogen, a tiny nucleus, converts about 0.3% of its mass into energy when it fuses with other hydrogen nuclei. Kilogram for kilogram, nuclear reactions release about 10 million times more energy than chemical reactions. Fortunately, they're much harder to start.
With that scientific background, let's follow a sequence of discoveries near the start of the twentieth century. Natural radioactive decay was discovered accidentally by French physicist Antoine Henri Becquerel (1852–1908) in 1896. Intrigued by the recent discovery of X-rays, he began looking for materials that might emit X-rays after exposure to light. To his surprise, he found that uranium fogged photographic plates, even through an opaque shield and even without exposure to light. His discovery was soon confirmed and elaborated on by Polish-born French physicist Marie Curie (1867–1934) and French chemist Pierre Curie (1859–1906). This wife and husband team discovered several new radioactive elements, including polonium (named after Marie's homeland) and radium.
In 1911, British physicist Ernest Rutherford (1871–1937) discovered that atoms have nuclei. He subsequently found that nuclei sometimes shatter when struck by energetic helium nuclei. In 1932, British physicist James Chadwick (1891–1974) discovered a fragment of the nucleus, the neutron, that has no electric charge and can thus approach a nucleus without any electrostatic repulsion. It was soon discovered that neutrons stick to the nuclei of many atoms.
The crucial discovery that made the atomic bomb possible was neutron-induced fission of nuclei. In 1934, Italian physicist Enrico Fermi (1901–1954) and his colleagues were trying to solve a particular riddle about the nucleus, a radioactive decay process called beta decay. They were adding neutrons to the nuclei of every atom they could get hold of. When they added neutrons to uranium, with its huge nucleus, they observed the production of some very short-lived radioactive systems. They thought that they had formed ultraheavy nuclei and even went so far as to give these new elements tentative names.
Four years later, however, Austrian physicists Lise Meitner (1878–1968) and Otto Frisch (1904–1979) and German chemists Otto Hahn (1879–1968) and Fritz Strassmann (1902–1980) collectively showed that what Fermi's group had actually done was to fragment uranium into lighter nuclei. Many of the fragments created by this induced fission were neutrons, which could themselves cause the destruction of other uranium nuclei.
Chain Reactions and the Fission Bomb
Physicists quickly realized that a chain reaction was possible, a reaction in which the fission of one uranium nucleus would induce fission in two nearby uranium nuclei, which would in turn induce fission in four other uranium nuclei, and so on. The result would be a catastrophic nuclear process in which many or even most of the nuclei in a piece of uranium would shatter and release fantastic amounts of energy.
In a sense, the remaining work toward both the atomic bomb and the hydrogen bomb was a matter of technical details. Only four conditions had to be satisfied for an atomic or fission bomb to be possible:
- A source of neutrons had to exist in the bomb to trigger the explosion.
- The nuclei making up the bomb had to be fissionable; that is, they had to fission when hit by a neutron.
- Each induced fission had to produce more neutrons than it consumed.
- The bomb had to use the released neutrons efficiently so that each fission induced an average of more than one subsequent fission.
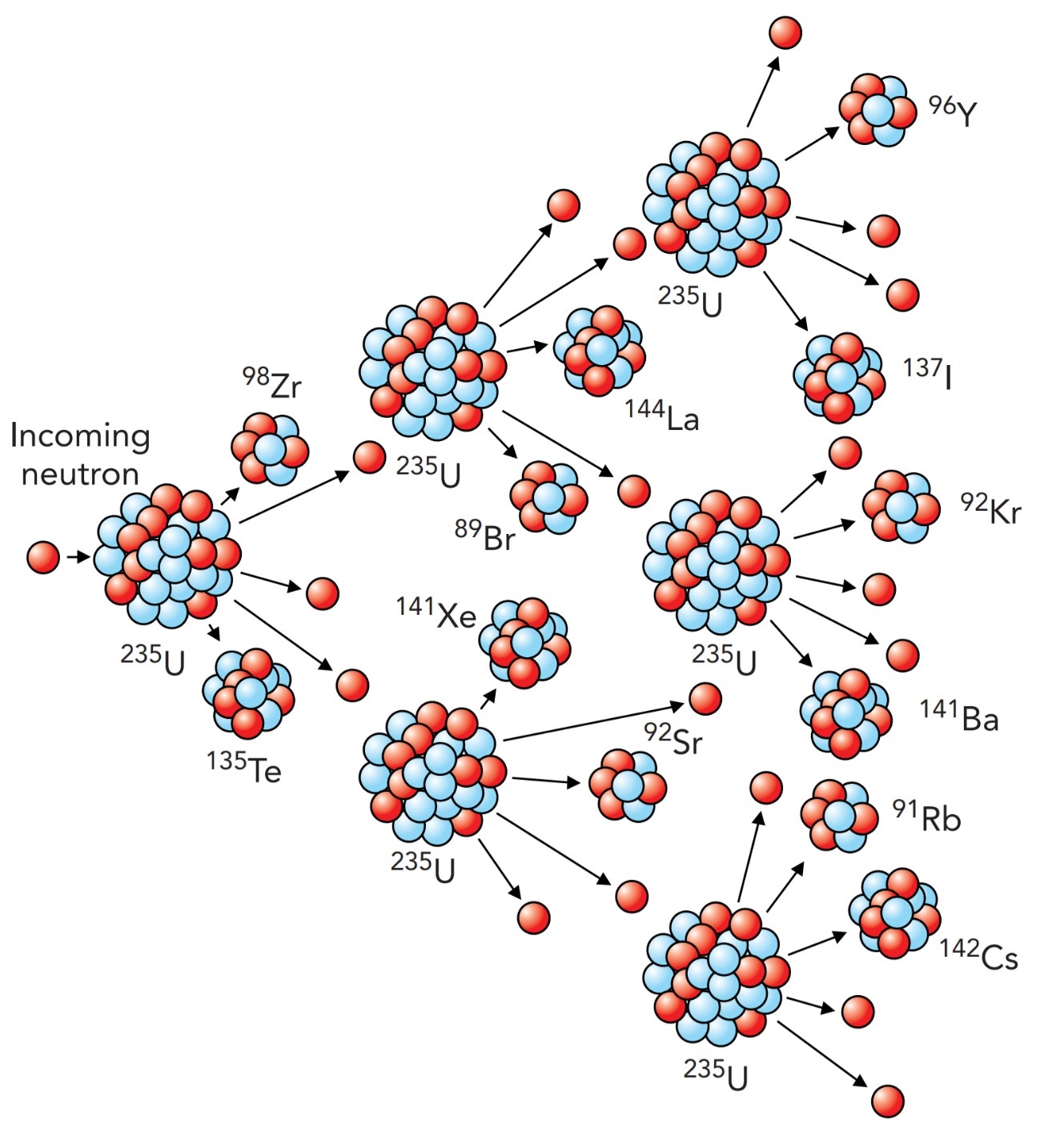
Meeting the first condition was easy. Many radioactive elements emit neutrons. Meeting the second and third conditions was more difficult. Here is where uranium fit into the picture. It was known to be fissionable, and it was known to release more neutrons than it consumed.
However, not all uranium nuclei are the same. Although a uranium nucleus must contain 92 protons, so that it forms a neutral atom with 92 electrons and has all the chemical characteristics of uranium, the number of neutrons in that nucleus is somewhat flexible. Nuclei that differ only in the numbers of neutrons they contain are called isotopes, and natural uranium nuclei come in two isotopes: Uranium 235 and Uranium 238, where the number specifies how many nucleons are in each nucleus. The Uranium 235 nucleus contains 92 protons and 143 neutrons, for a total of 235 nucleons. In contrast, the Uranium 238 nucleus contains 238 nucleons: 92 protons and 146 neutrons.
It turns out that only Uranium 235 is suitable for a bomb. It's marginally stable, with too many protons for the nuclear force to keep together, even with the diluting effects of 143 neutrons. Like many proton-rich nuclei, Uranium 235 eventually undergoes alpha decay; it emits a helium nucleus (4 He) and thereby loses two protons and two neutrons. Uranium 235 has a radioactive half life of 710 million years. However, when struck by a neutron, Uranium 235 shatters immediately into fragments, and this induced fission releases about 2.5 neutrons.
Uranium 238 is slightly more stable than the lighter isotope, and its half-life is 4.51 billion years. The Uranium 238 nucleus absorbs most neutrons without undergoing fission. Instead, it undergoes a series of complicated nuclear changes that eventually convert it into plutonium, an element not found in nature. It becomes Plutonium 239, which has a nucleus with 94 protons and 145 neutrons. Plutonium itself is useful for making nuclear weapons.
Thus Uranium 238 actually slows a chain reaction rather than encouraging it. Since only Uranium 235 can support a chain reaction, natural uranium has to be separated before it can be used in a bomb. Uranium 235 is quite rare, however. Earth's store of uranium nuclei was created long ago, in the explosion of a dying star. That supernova heated the nuclei of smaller atoms so hot that they collided together and stuck. Uranium nuclei were formed, with the supernova's energy trapped inside them. They were incorporated in the Earth during its formation about 4 or 5 billion years ago and have been decaying ever since. The only uranium isotopes that remain in any quantity are Uranium 235 and Uranium 238. Since Uranium 235 is less stable, its percentage of the naturally occurring uranium nuclei has dwindled to only 0.72%. The remaining 99+% of the uranium is Uranium 238.
Separating Uranium 235 from Uranium 238 is extremely difficult. Since atoms containing these two nuclei differ only in mass, not in chemistry, they can be separated only by methods that compare their masses. Because the mass difference is relatively small, heroic measures are needed to extract Uranium 235 from natural uranium. During World War II and the Cold War era, the U.S. government developed enormous facilities for separating the two uranium isotopes. The need for such installations is one of the major obstacles to the proliferation of nuclear weapons.
The last condition for sustaining a chain reaction is that the bomb must use neutrons efficiently, so that each fission induces an average of more than one subsequent fission. That means that the bomb's contents can't absorb neutrons wastefully and that it can't let too many of them escape without causing fission. A lump of relatively pure Uranium 235 wouldn't absorb neutrons wastefully, but it might allow too many of them to escape through its surface. For a chain reaction to occur, the lump must be large enough that each neutron has a good chance of hitting another nucleus before it leaves the lump. The lump also should have a minimal amount of surface. It should be a sphere.
How large must that sphere be? Since atoms are mostly empty space, a neutron can travel several centimeters through a lump of uranium without hitting a nucleus. Thus a golf ball–size sphere of uranium would allow too many neutrons to escape. For a bare sphere of Uranium 235, the critical mass required to initiate a chain reaction is about 52 kg, a ball about 17 cm in diameter. At that point, each fission will induce an average of one subsequent fission. For an explosive chain reaction, in which each fission induces an average of much more than one fission, additional Uranium 235 is needed—a supercritical mass. About 60 kg will do it.
By 1945, the scientists and engineers of the Manhattan Project had found ways to meet these four conditions and were prepared to initiate an explosive chain reaction. They had accumulated enough Uranium 235 to construct a supercritical mass. Carefully machined pieces of Uranium 235 would be put into the bomb so that they would join together at the moment the bomb was to explode. When the critical mass was reached, a few initial neutrons would start the chain reaction. When the uranium became supercritical, catastrophic fission would quickly turn it into a tremendous fireball.
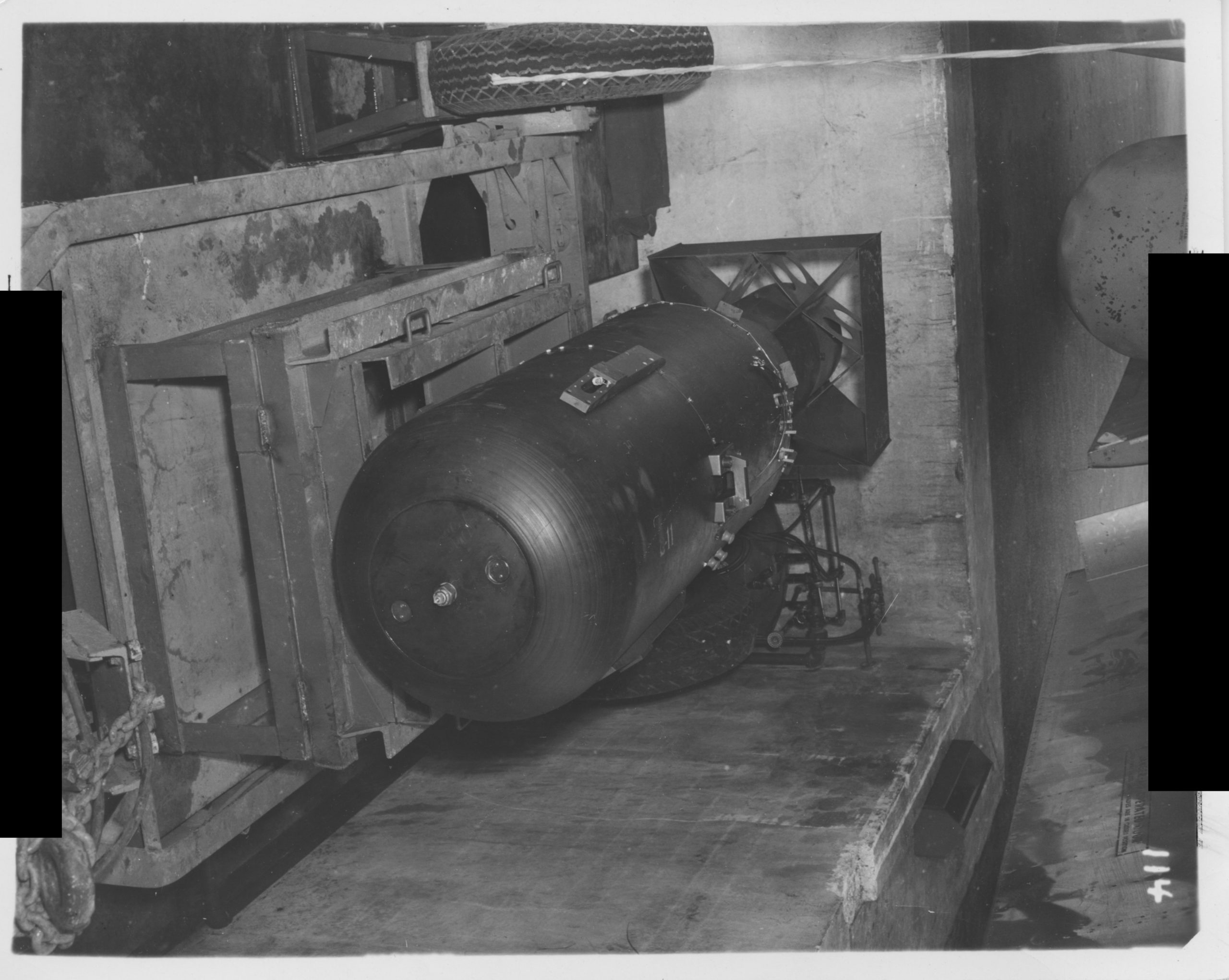
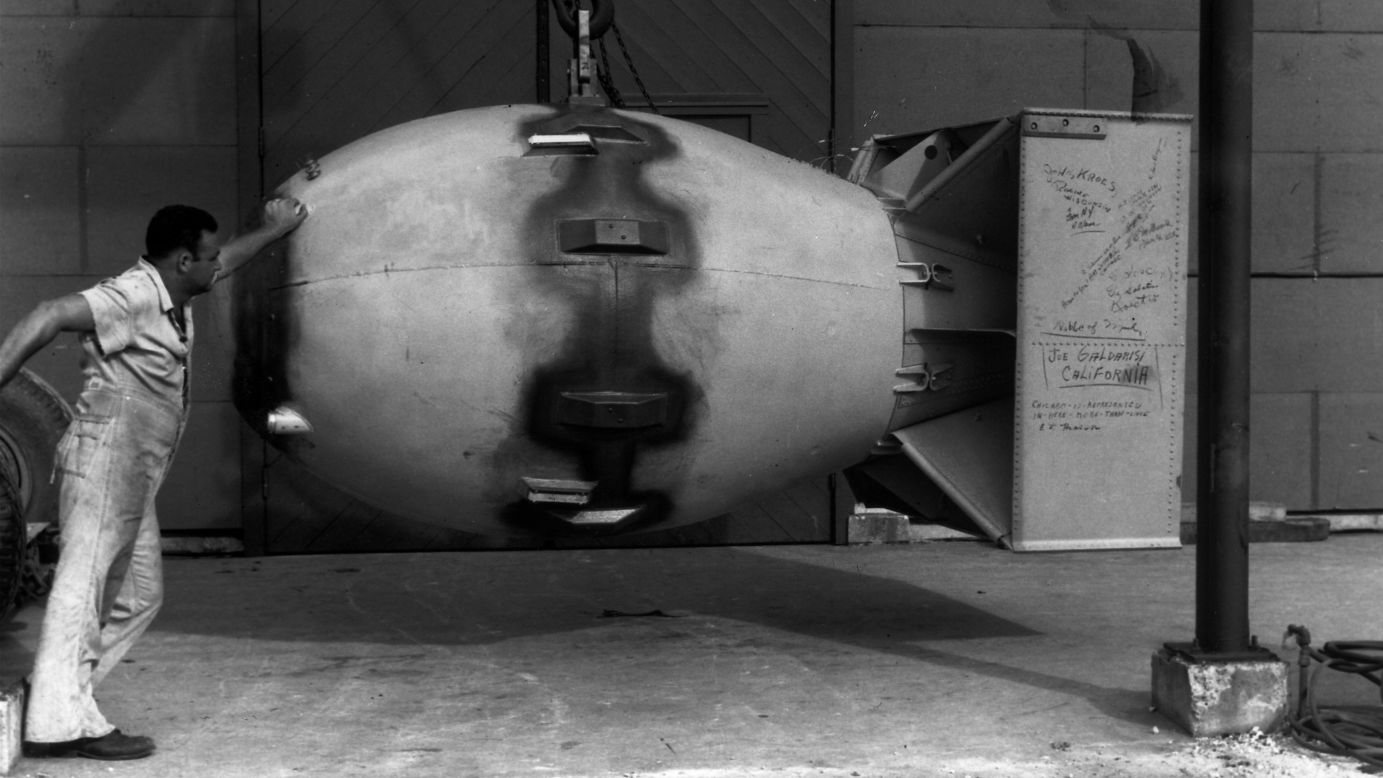
However, assembly was tricky. The supercritical mass had to be completely assembled before the chain reaction was too far along; otherwise the bomb would begin to overheat and explode before enough of its nuclei had time to fission. In pure Uranium 235, the time it takes for one fission to induce the next fission is only about 10 nanoseconds or 10 billionths of a second. In a supercritical mass, each generation of fissions is much larger than the previous generation, so it takes only a few dozen generations to shatter a significant fraction of the uranium nuclei. The whole explosive chain reaction is over in less than a millionth of a second, with most of the energy released in the last few generations about 30 nanoseconds.
To make sure that the assembly was complete before the bomb exploded, it had to be done extraordinarily quickly. For the Uranium 235 bomb called Little Boy that was exploded over Hiroshima, Japan, on August 6, 1945, at 8:15 am and was responsible for the deaths of about 200,000 Japanese citizens, the supercritical mass was assembled when a cannon fired a cylinder of Uranium 235 through a hole in a sphere of Uranium 235. When the cylinder was centered in the hole, it completed a 60 kg sphere of uranium, housed in a tungsten carbide and steel container. This container confined the uranium, holding it together with its inertia as the explosion began. An explosive chain reaction started immediately, and by the time the uranium blew itself apart, 1.3% of the Uranium 235 nuclei had fissioned. The energy released in that event was equal to the explosion of about 15,000 tons of TNT.
But Little Boy was actually the second nuclear explosion. Its concept was so foolproof and its Uranium 235 so precious that Little Boy was dropped without ever being tested. However, the Manhattan Project had also developed a plutonium-based bomb that involved a much more sophisticated concept. This bomb was much less certain to work, so it was tested once before it was used.
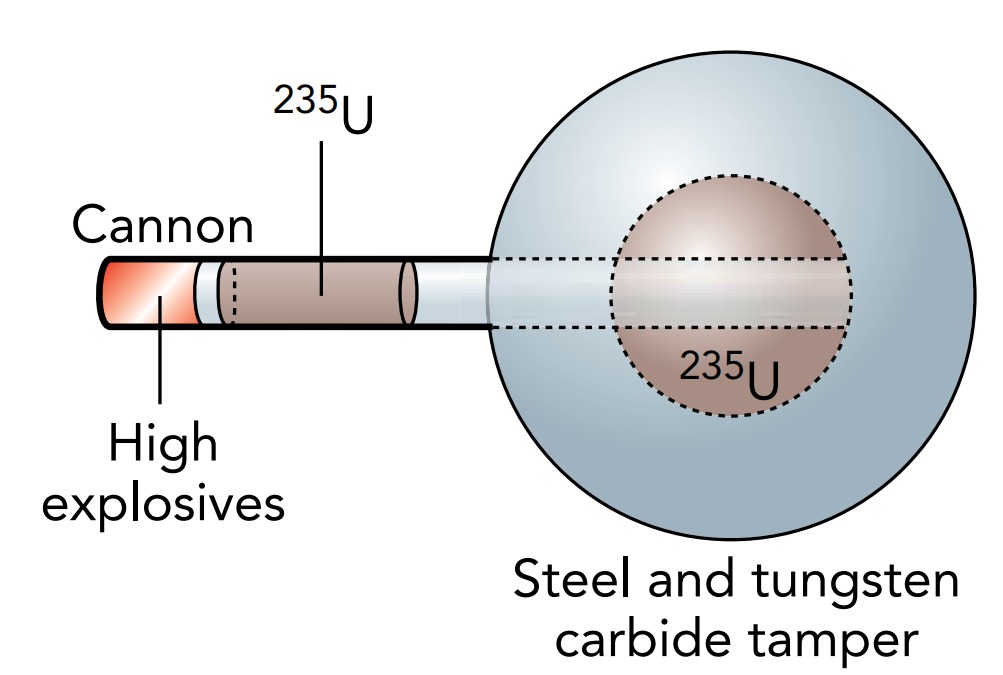
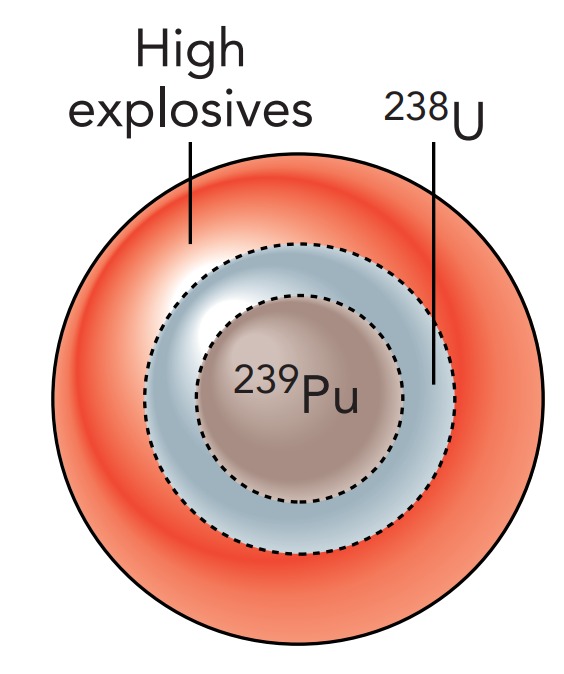
The Gadget, as the first atomic bomb was called, didn't use Uranium 235. Instead, it used plutonium that had been synthesized from Uranium 238 in nuclear reactors. A nuclear reactor carries out a controlled chain reaction in uranium, and neutrons from this chain reaction can convert Uranium 238 into Plutonium 239.
Like Uranium 235, Plutonium 239 meets the conditions for a bomb. The Plutonium 239 nucleus is relatively unstable, with a half-life of only 24,400 years. It fissions easily when struck by a neutron and releases an average of three neutrons when it does. Thus, Plutonium 239 can be used in a chain reaction. For a bare sphere of Plutonium 239, the critical mass is about 10 kg - a ball about 10 cm in diameter.
There is a problem with Plutonium 239, however. It's so radioactive and releases so many neutrons when it fissions that a chain reaction develops almost instantly. There is much less time to assemble a supercritical mass of plutonium than there is with uranium. The cannon assembly method won't work because the plutonium will overheat and blow itself apart before the cylinder can fully enter the sphere.
Thus a much more sophisticated assembly scheme was employed for the Gadget. At 5:29 am on June 16, 1945, over 2000 kg of carefully designed high explosives crushed or imploded a sphere of plutonium inside the Gadget at Alamogordo, New Mexico. By itself, the 6.1 kg sphere wasn't large enough to be a critical mass; it was a subcritical mass. However, it was surrounded by a tamper of Uranium 238 whose massive nuclei reflected many neutrons back into the plutonium like marbles bouncing off bowling balls. The implosion process compressed the plutonium well beyond its normal density. With the plutonium nuclei packed more tightly together, they were more likely to be struck by neutrons and undergo fission.
The scheme worked. The chain reaction that followed caused 17% of the plutonium nuclei to fission and released energy equivalent to the explosion of about 22,000 tons of TNT. The tower and equipment at the Trinity test site disappeared into vapor, and the desert sands turned to glass for hundreds of meters in all directions. A nearly identical device named Fat Man was dropped over Nagasaki, Japan, on August 9, 1945, at 11:02 am, where it ultimately killed about 140,000 people.
In the years following the first fission bombs, development focused on how best to bring the fissionable material together. The longer a supercritical mass could be held together before it overheated and exploded, the larger would be the fraction of its nuclei that would fission and the greater the explosive yield. The crushing technique of The Gadget and Fat Man became the standard, and bombs grew smaller and more efficient at using their nuclear fuel. The implosion process reduced the amount of plutonium needed to reach a supercritical mass, so very small fission bombs were possible. The smallest atomic bomb, the Davy Crockett, weighed only about 220 N.
The Fusion or Hydrogen Bomb
Since fissionable material begins to explode as soon as it exceeds critical mass, this critical mass limits the size and potential explosive yield of a fission bomb. In search of a way around this limit, bomb scientists took a look back at small nuclei and figured out how to extract energy by sticking them together.
The fission bomb brought to Earth, for the first time, temperatures that had previously been observed only in stars. Stars obtain most of their energy by fusing hydrogen nuclei together to form helium nuclei, a process that releases a great deal of energy. Because hydrogen nuclei are protons and repel one another with tremendous forces, hydrogen doesn't normally undergo fusion here on Earth. To cause fusion, something must bring those protons close enough for the nuclear force to stick them together. The only practical way we know of to bring the nuclei together is to heat them so hot that they crash into each other. That is what happens in a fusion bomb, also called a thermonuclear or hydrogen bomb.
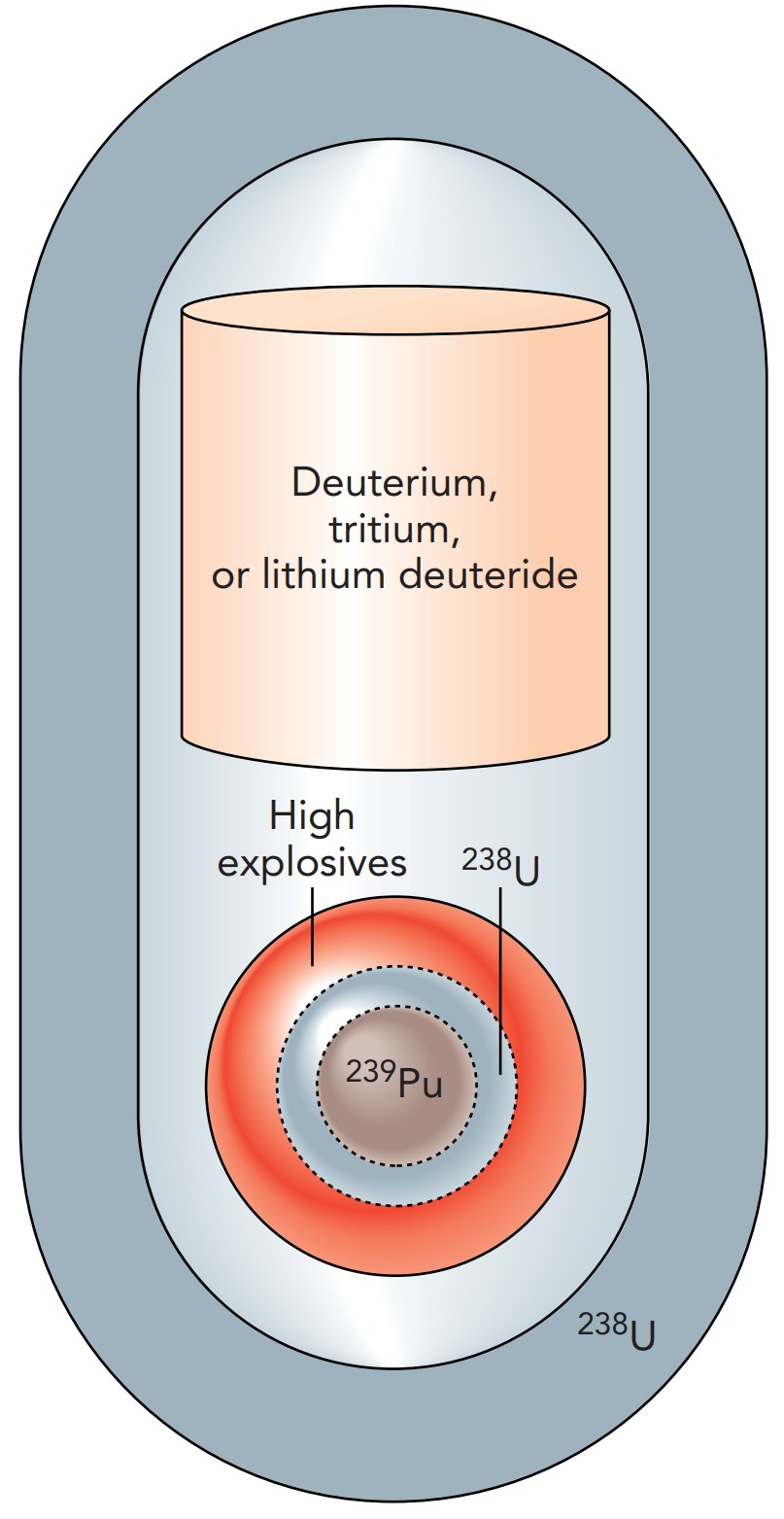
In a fusion bomb, an exploding fission bomb heats a quantity of hydrogen to about 100 million degrees Celsius. At that temperature, hydrogen nuclei begin to collide with one another. To ease the nuclear fusion processes, heavy isotopes of hydrogen are used: deuterium (2 H) and tritium (3 H). While the normal hydrogen nucleus (1 H) contains only a proton, the deuterium nucleus contains a proton and a neutron. The tritium nucleus contains a proton and two neutrons. When a deuterium nucleus collides with a tritium nucleus, they stick to form a helium nucleus (4 He) and a free neutron. Because this process converts about 0.3% of the original mass into energy, the helium nucleus and the neutron fly away from one another at enormous speeds.
Since hydrogen won't explode spontaneously, even in huge quantities, a hydrogen bomb can be extremely large. A fission bomb is used to set it off, but after that, the sky's the limit. Some enormous fusion bombs were constructed and tested during the early days of the Cold War. These bombs usually consisted of a fission starter and a hydrogen follower, all wrapped up in a tamper of Uranium 238. The tamper confined the hydrogen as the fusion began. Once fusion was underway, converting deuterium and tritium into helium and neutrons, the neutrons collided with Uranium 238 nuclei in the tamper. These fusion neutrons were so energetic that they were able to induce fissions even in Uranium 238 nuclei and release still more energy. Overall, this structure is sometimes called a fission fusion fission bomb.
A variation on this bomb is the so-called neutron or enhanced radiation bomb. That bomb has no Uranium 238 tamper, so the energetic neutrons from the fusion process travel out of the explosion and irradiate everything in the vicinity. This bomb is lethal to humans, but because its blast is relatively weak, it is not particularly destructive to property.
Tritium is a radioactive isotope created in nuclear reactors. It has too many neutrons to be a stable nucleus and slowly decays into a light isotope of helium (3 He). Because tritium has a half-life of 12.3 years, fusion bombs containing tritium require periodic maintenance to replenish their tritium.
Many fusion bombs use solid lithium deuteride instead of deuterium and tritium gases. Lithium deuteride is a salt containing lithium (6 Li) and deuterium (2 H). When a neutron from the fission starter collides with a 6 Li nucleus, the two fragment into a helium nucleus (4 He) and a tritium nucleus (3 H). In the bomb, lithium deuteride is quickly converted into a mixture of deuterium, tritium, and helium, which then undergoes fusion.
Aftermath of Nuclear explosion
Once a nuclear weapon has exploded—after its fissionable material has fissioned and its fusible material has fused—what then? First, a vast number of nuclei and subatomic particles emerge from the explosion at enormous speeds, many at nearly the speed of light. These particles crash into nearby atoms and molecules, heating them to fantastic temperatures and producing a local fireball around the bomb itself. They also cause extensive radiation damage in the surrounding area.
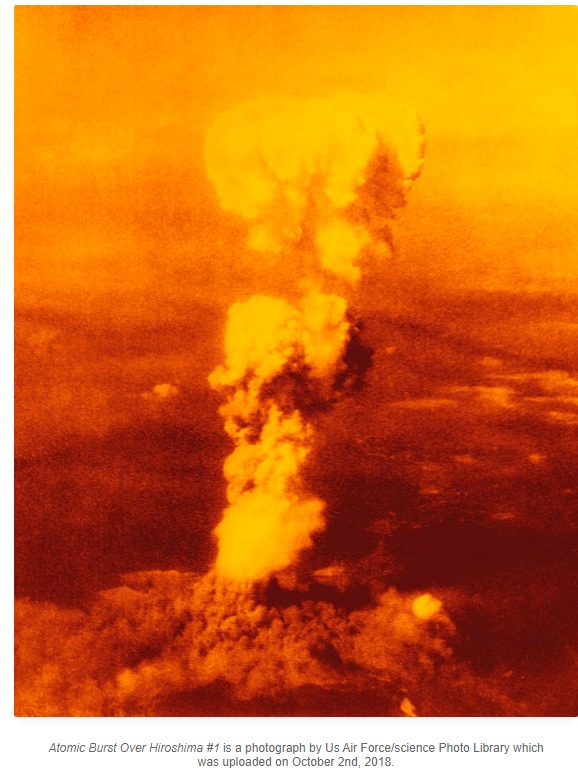
Second, a flash of light emerges from the explosion, caused partly by the fission and fusion processes themselves and partly by the ultrahot fireball that follows. This light is not only visible light, but also every portion of the electromagnetic spectrum from infrared to visible to ultraviolet to X-rays to gamma rays. It burns things nearby, inside and out. Third, the explosion creates a huge pressure surge in the air around the fireball. A shock wave propagates outward from the fireball at the speed of sound, knocking over everything in its path for a considerable distance. Fourth, the rarefied and superheated air rushes upward, lifted by buoyant forces, to create a towering mushroom cloud.
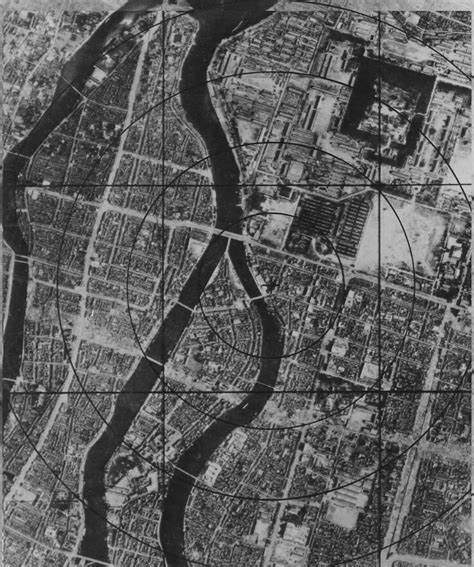
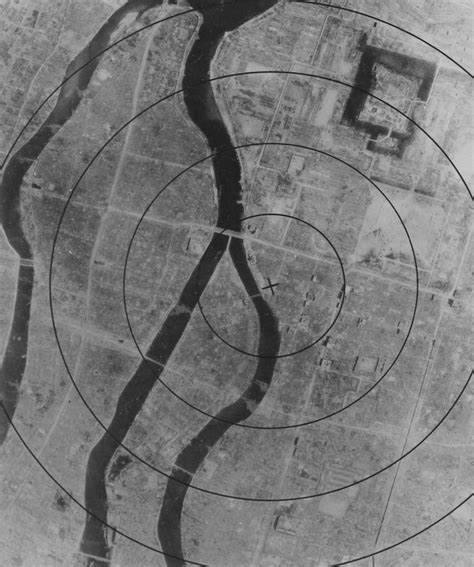
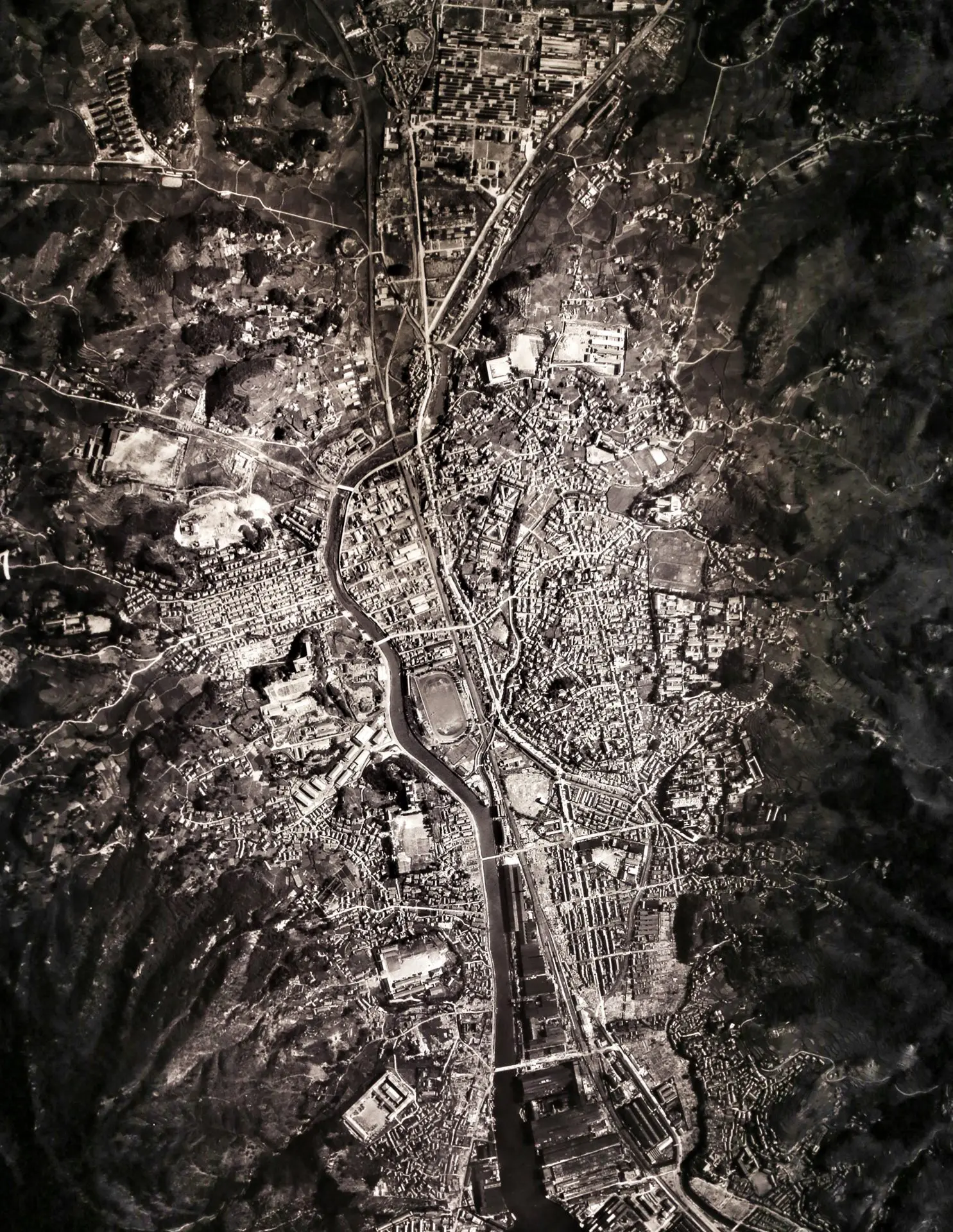
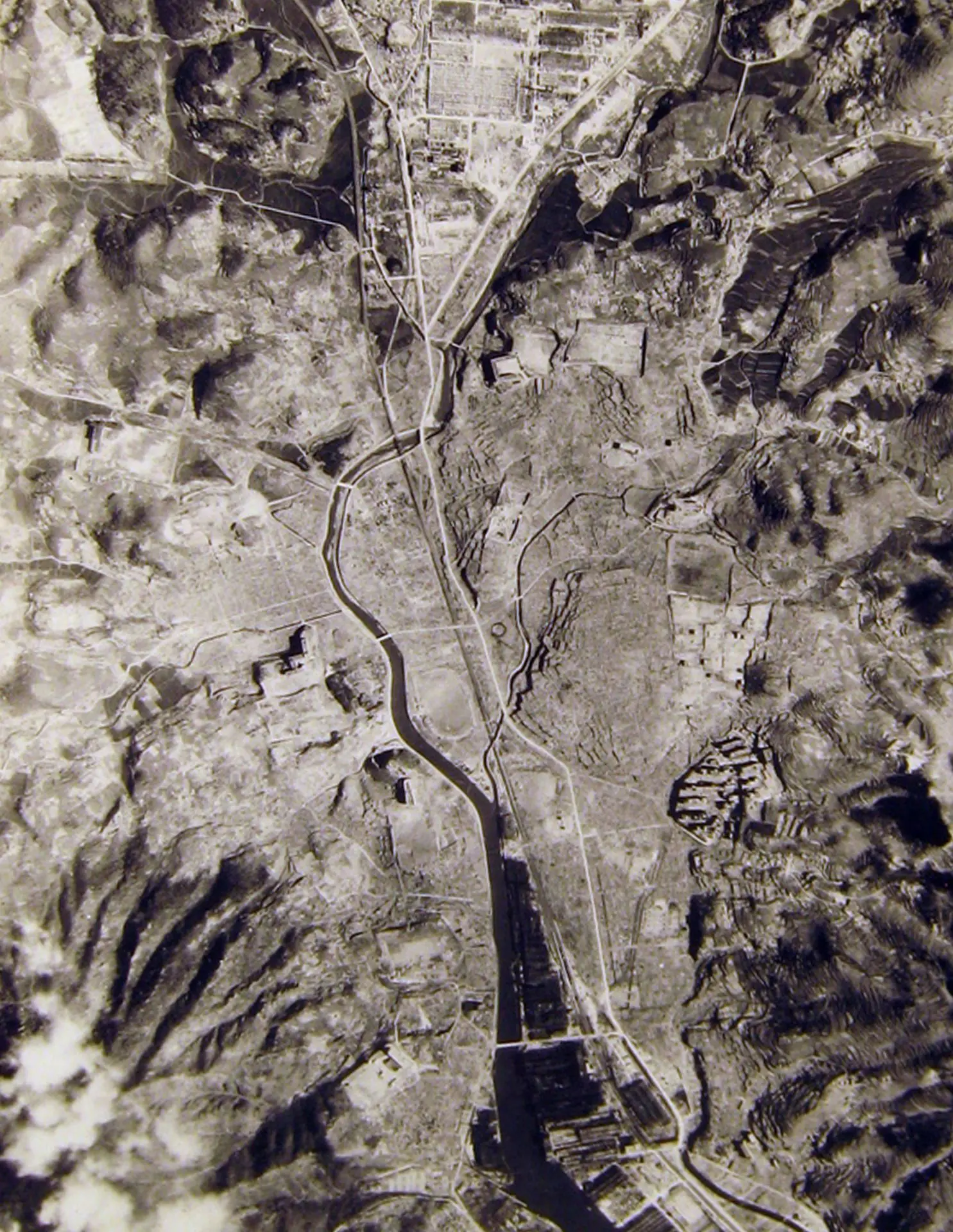
However, the most insidious aftereffect of a nuclear explosion is fallout, the creation and release of radioactive nuclei. Fission converts uranium and plutonium nuclei into smaller nuclei. Each new nucleus has several dozen protons and its share of neutrons from the nucleus that fissioned. These new nuclei attract electrons and become seemingly normal atoms like iodine or cobalt. But while large nuclei such as uranium need extra neutrons to dilute their protons and reduce their electrostatic repulsions, intermediate and small nuclei like those of iodine and cobalt don't need as many neutrons. The new nuclei wind up with too many neutrons and are radioactive. They have half-lives that are anywhere from thousandths of a second to thousands of years. Until they decay, the atoms that contain these nuclei are almost indistinguishable from normal atoms. They are radioactive isotopes of common atoms, and our bodies naively incorporate them into our tissues. There they sit, performing whatever chemical tasks our bodies require of them. Eventually, however, these radioactive atoms fall apart and release nuclear energy.
Because each radioactive decay that occurs near us or inside us releases perhaps a million times more energy than is present in a chemical bond, these decays cause chemical changes in our cells. They can kill cells or damage the cells' genetic information, potentially causing cancer.
This transmutation of elements, the restructuring of nuclei to transform atoms of one element into another, occurs in an uncontrolled fashion in nuclear weapons and produces a lethal mix of unstable isotopes. Those isotopes take years to decay out of the environment, and all anyone can do is wait. Even nuclear weapons with poor explosive yields, so-called dirty bombs, can litter the surrounding landscape with radioactive debris. Nuclear reactors produce similar mixtures of radioactive isotopes in their fuel assemblies and core structures, which is why disposing of spent nuclear fuel remains so problematic.
On the other hand, radioactive isotopes have been a boon to medicine and biochemistry, where many of them have found valuable and life-saving applications. Moreover, in controlled circumstances, elements can be transmuted systematically to generate primarily desirable isotopes rather than a random assortment. Such transmutation is difficult and expensive, and it involves nuclear rather than chemical processes. Although the alchemists' dream of transmuting lead into gold is finally possible, it's not a path to riches.