Nuclear Plants
Making weapons weren't the only possibilities open to nuclear scientists and engineers at the end of the 1930s. While nuclear fission chain reactions and thermonuclear fusion were clearly ways to unleash phenomenal destructive energy, they could also provide virtually limitless sources of useful energy. By controlling the same nuclear reactions that occur in nuclear weapons, people have since managed to extract nuclear energy for constructive uses. In the half century since their conception, nuclear fission reactors have developed into a fairly mature technology and have become one of our major sources of energy. Nuclear fusion power remains an elusive goal, but efforts continue to harness this form of nuclear energy as well. Competing concerns about nuclear safety, nuclear weapons proliferation, and climate change will no doubt influence the future of these technologies.
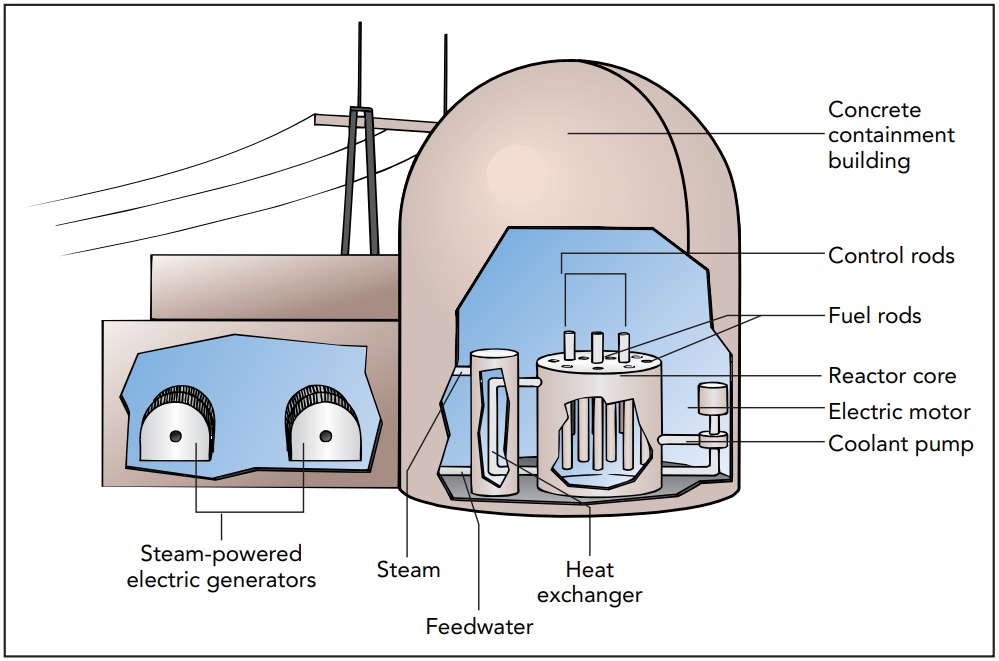
Nuclear Fission Reactors
Assembling a critical mass of uranium doesn't always cause a nuclear explosion. In fact, it's rather hard to cause a big explosion. The designers of the atomic bomb had to assemble not just a critical mass but a supercritical mass, and they had to do it in much less than a millionth of a second. That rapid assembly is not something that happens easily or by accident. It's much easier to reach a critical mass slowly, in which case the uranium will simply become very hot. It may ultimately explode from overheating, but it will not vaporize everything in sight.
This slow assembly of a critical mass is the basis for nuclear fission reactors. Their principal product is heat, which is usually used to generate electricity. Fission reactors are much simpler to build and operate than fission bombs because they don't require such purified fissionable materials. In fact, with the help of some clever tricks, nuclear reactors can be made to operate even with natural uranium.
Let's begin by showing that a fission chain reaction doesn't always lead to an explosion. What's important is just how fast the fission rate increases. In an atomic bomb, it increases breathtakingly quickly. At detonation, the fissionable material is far above the critical mass, so the average fission induces not just one but perhaps two subsequent fissions. With only about 10 nanoseconds between one fission and the two it induces, the fission rate may double every 10 nanoseconds. In less than a millionth of a second, most of the nuclei in the material undergo fission, releasing their energy before the material has time to blow apart.
However, things aren't as dramatic right at critical mass, where the average fission induces just one subsequent fission. Since each generation of fissions simply reproduces itself, the fission rate remains essentially constant. Only spontaneous fissions cause it to rise at all. The fissionable material steadily releases thermal energy, and that energy can be used to power an electric generator.
A nuclear reactor contains a core of fissionable material. Because of the way in which this core is assembled, it's very close to a critical mass. Several neutronabsorbing rods, called control rods, which are inserted into the reactor's core, determine whether it's above or below critical mass. Pulling the control rods out of the core increases the chance that each neutron will induce a fission and moves the core toward supercriticality. Dropping the control rods into the core increases the chance that each neutron will be absorbed before it can induce a fission and moves the core toward subcriticality.
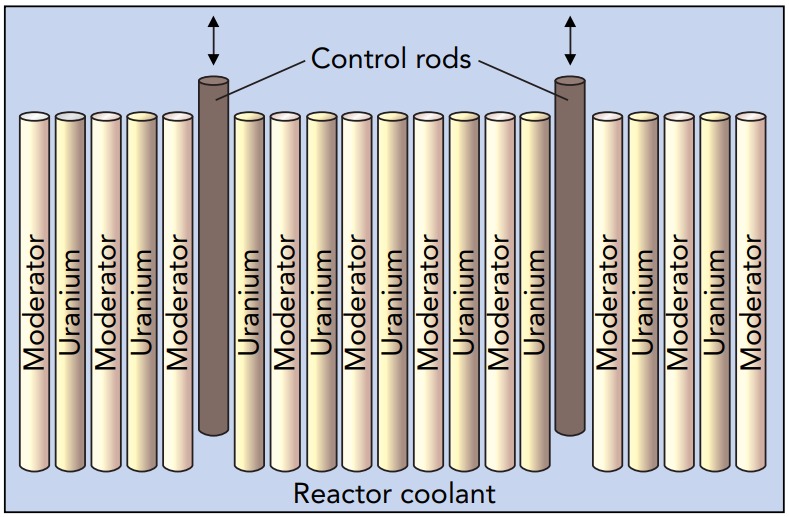
A nuclear reactor uses feedback to maintain the fission rate at the desired level. If the fission rate becomes too low, the control system slowly pulls the control rods out of the core to increase the fission rate. If the fission rate becomes too high, the control system drops the control rods into the core to decrease the fission rate. It's like driving a car. If you're going too fast, you ease off the gas pedal. If you are going too slowly, you push down on the gas pedal.
The car-driving analogy illustrates another important point about reactors. Both cars and reactors respond relatively slowly to adjustments of their controls. It would be hard to drive a car that stopped instantly when you lifted your foot off the gas pedal and leaped to supersonic speed when you pushed your foot down. Similarly, it would be impossible to operate a reactor that immediately shut down when you dropped the control rods in and that instantly exploded when you pulled the control rods out.
But reactors, like cars, don't respond quickly to movements of the control rods. That's because the final release of neutrons following some fissions is slow. When a Uranium 235 nucleus fissions, it promptly releases an average of 2.47 neutrons which induce other fissions within a thousandth of a second. However, some of the fission fragments are unstable nuclei that decay and release neutrons long after the original fission. On average, each Uranium 235 fission eventually produces 0.0064 of these delayed neutrons, which then go on to induce other fissions. It takes seconds or minutes for these delayed neutrons to appear, and they slow the response of the reactor. The reactor's fission rate can't increase quickly because it takes a long time for the delayed neutrons to build up. The fission rate can't decrease quickly because it takes a long time for the delayed neutrons to go away.
To further ease the operation of modern nuclear reactors, they are designed to be stable and self-regulating. This self-regulation ensures that the core automatically becomes subcritical if it overheats. This self-regulation was not present in the design of Chernobyl Reactor Number 4 and that led to disaster.
Thermal Fission Reactors
The basic concept of a nuclear reactor is simple: assemble a critical mass of fissionable material and adjust its criticality to maintain a steady fission rate. But what should the fissionable material be? In a fission bomb, it must be relatively pure Uranium 235 or Plutonium 239. In a fission reactor, however, it can be a mixture of Uranium 235 and Uranium 238. It can even be natural uranium. The trick is to use thermal neutrons, slow-moving neutrons that have only the kinetic energy associated with the local temperature.
In a fission bomb, Uranium 238 is a serious problem because it captures the fast-moving neutrons emitted by fissioning Uranium 235 nuclei. Natural uranium alone can't sustain a chain reaction because its many Uranium 238 nuclei gobble up most of the fast-moving neutrons before they can induce more fissions in the rare Uranium 235 nuclei. The uranium must be highly enriched—most of its Uranium 238 nuclei must be removed so that it contains far more than the natural abundance of Uranium 235.
Slow-moving neutrons have a different experience as they travel through natural uranium. For complicated reasons, the Uranium 235 nuclei seek out slow-moving neutrons and capture them with remarkable efficiency. Uranium 235 nuclei are so good at catching slow-moving neutrons that they easily win out over the more abundant Uranium 238 nuclei. Even in natural uranium, a slow-moving neutron is more likely to be caught by a Uranium 235 nucleus than it is by a Uranium 238 nucleus. As a result, it's possible to sustain a nuclear fission chain reaction in natural uranium if all of the neutrons are slow moving.
Because Uranium 235 nuclei emit fast-moving neutrons when they fission, natural or slightly enriched uranium alone can't use the slow-moving-neutron effect to maintain a chain reaction. However, most nuclear reactors contain something else besides uranium, natural or otherwise. Along with uranium, they use a material called a moderator that slows the neutrons down so that Uranium 235 nuclei can grab them. A fast-moving neutron from a fissioning Uranium 235 nucleus enters the moderator, rattles around for about a thousandth of a second, and emerges as a slow-moving neutron, one with only thermal energy left. It then induces fission in another Uranium 235 nucleus. Once the moderator is present, even natural uranium can sustain a chain reaction! Reactors that carry out their chain reactions with slow-moving, or thermal, neutrons are called thermal fission reactors.
To be a good moderator, a material must remove energy and momentum from the neutrons without absorbing them. A fast-moving fission neutron entering that moderator should leave with only thermal energy. The best moderators are small nuclei that rarely or never absorb neutrons and don't fall apart during collisions with them. Hydrogen (1H), deuterium (2H), helium (4He), and carbon (12C) are all good moderators. When a fastmoving neutron hits the nucleus of one of these atoms, the collision resembles that between two bumper cars. Because the fast-moving neutron transfers some of its energy and momentum to the nucleus, the neutron slows down while the nucleus speeds up. That collision is most effective when the neutron and nucleus have similar masses, so small nuclei are better moderators than large ones.
Water, heavy water, that is, water containing the heavy isotope of hydrogen, deuterium, and graphite or carbon are the best moderators for nuclear reactors. They slow neutrons down to thermal speeds without absorbing many of them. Of these moderators, heavy water is the best because it slows the neutrons quickly yet doesn't absorb them at all. However, heavy water is expensive because only 0.015% of hydrogen atoms are deuterium and separating that deuterium from ordinary hydrogen is difficult. Graphite moderators were used in many early reactors because graphite is cheap and working with it is easy. However, graphite is a less efficient moderator than heavy water, so graphite reactors had to be big. Furthermore, graphite can burn and was partly responsible for two of the world's four major reactor accidents. Normal, or “light,” water is cheap, safe, and an efficient moderator, but it absorbs enough neutrons that it can't be used with natural uranium. For use in a light-water reactor, uranium must be enriched slightly, to about 2–3% Uranium 235.
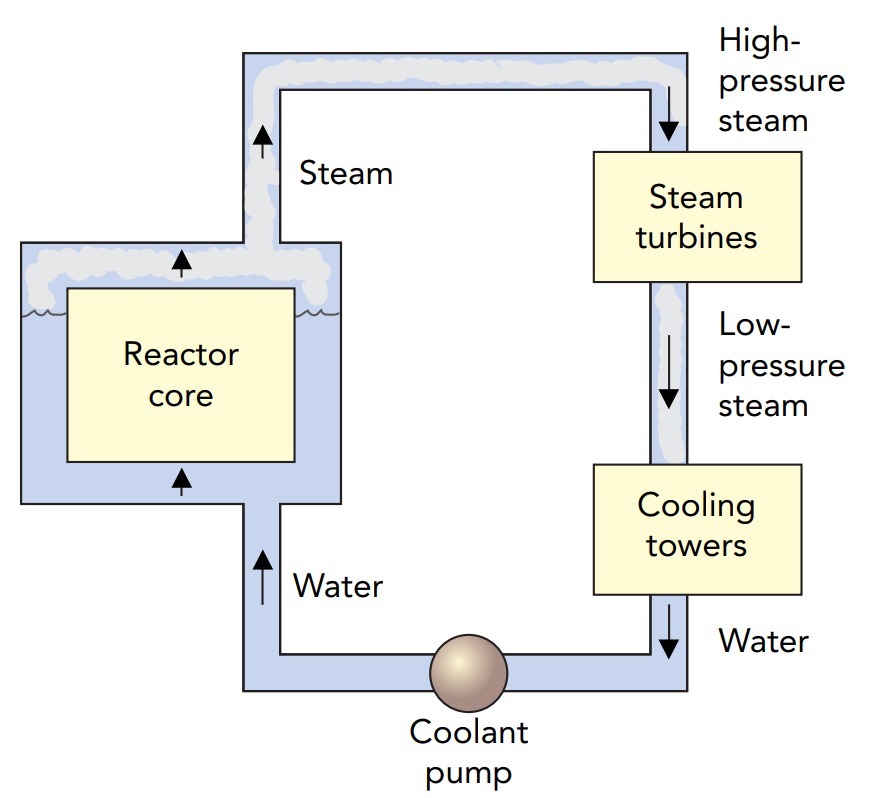
The core of a typical thermal fission reactor consists of small uranium oxide fuel pellets separated by layers of moderator. A neutron released by a fissioning Uranium 235 nucleus usually escapes from its fuel pellet, slows down in the moderator, and then induces fission in a Uranium 235 nucleus in another fuel pellet. By absorbing some of these neutrons, the control rods determine whether the whole core is subcritical, critical, or supercritical. The Uranium 238 nuclei are basically spectators in the reactor since most of the fissioning occurs in the Uranium 235 nuclei.
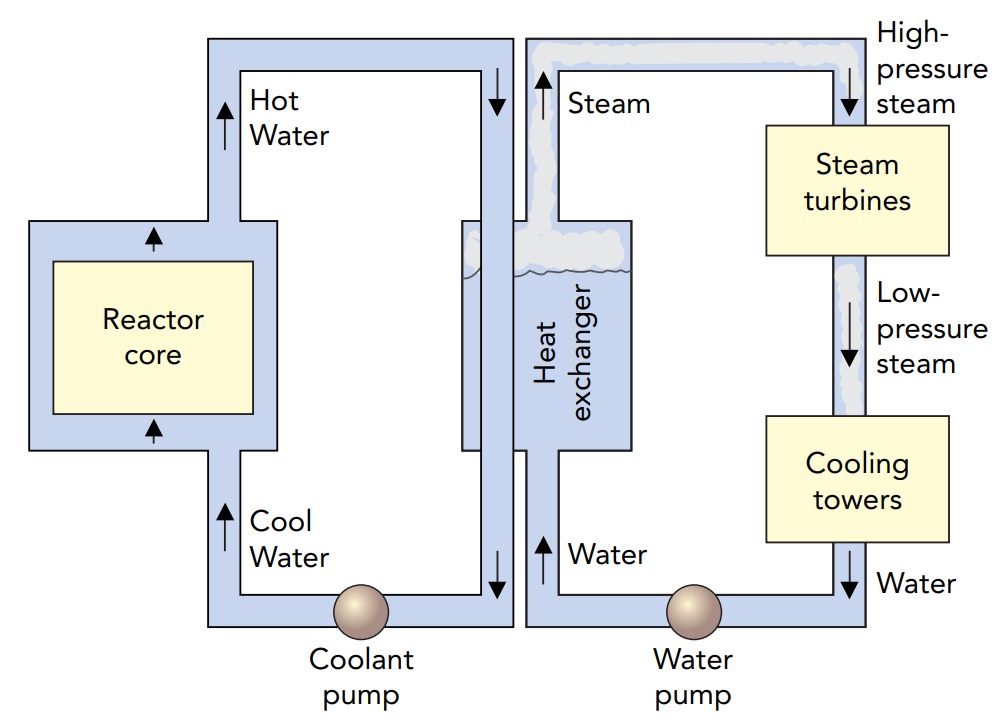
In a practical thermal fission reactor, something must extract the heat released by nuclear fission. In many reactors, cooling water passes through the core at high speeds. Heat flows into this water and increases its temperature. In a boiling water reactor, the water boils directly in the reactor core, creating high-pressure steam that drives the turbines of an electric generator. In a pressurized water reactor, the water is under enormous pressure, so it can't boil. Instead, it's pumped to a heat exchanger outside the reactor. This heat exchanger transfers heat to water in another pipe, which boils to create the high-pressure steam that drives a generator.
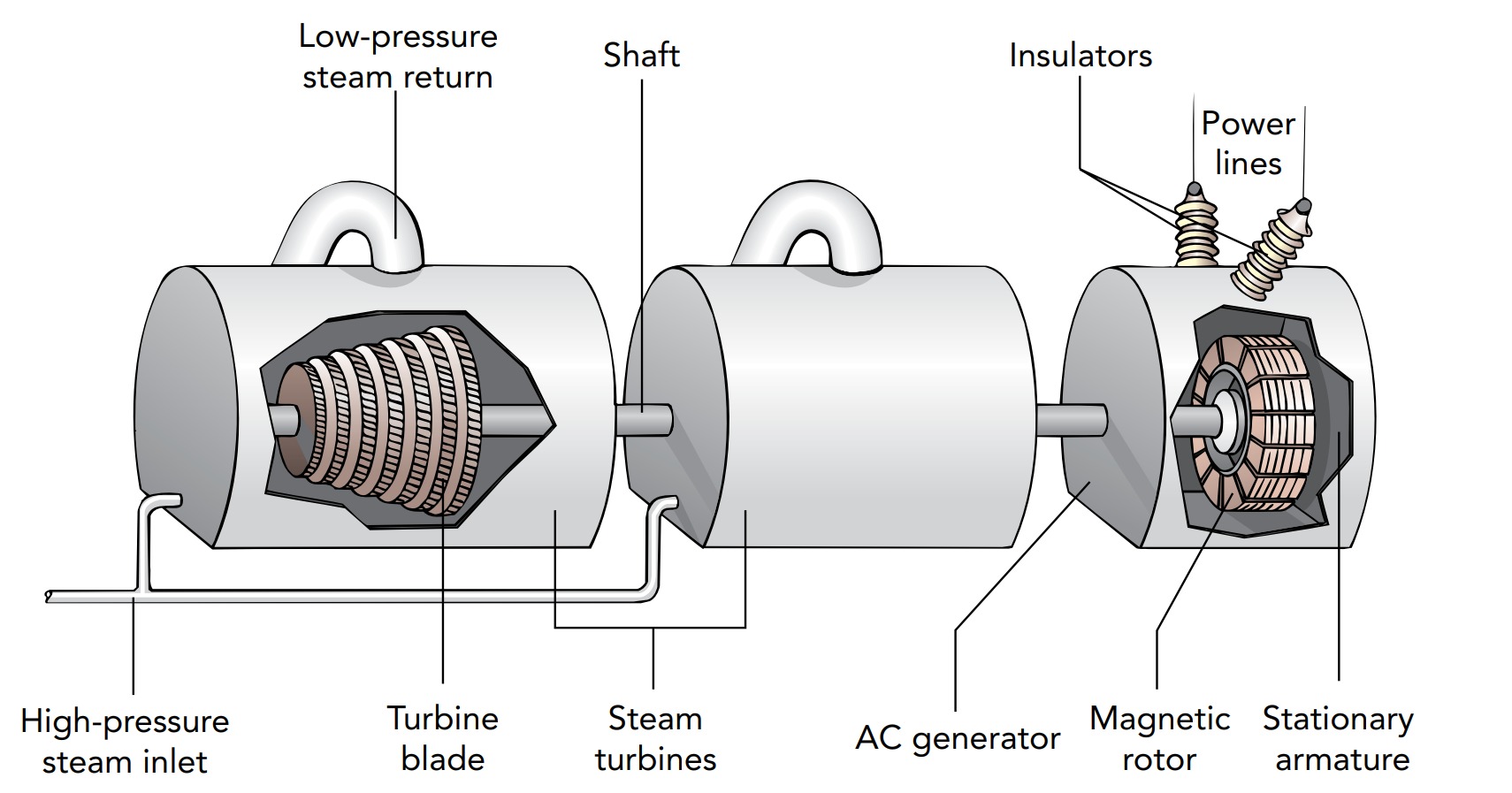
When properly designed, a water-cooled thermal fission reactor is inherently stable. The cooling water is actually part of the moderator. If the reactor overheats and the water escapes, there will no longer be enough moderator around to slow the fission neutrons down. The fast-moving neutrons will be absorbed by Uranium 238 nuclei, and the chain reaction will slow or stop.
Fast Fission Reactors
Thermal reactors require simple fuel and are relatively straightforward to construct. However, they consume only the Uranium 235 nuclei and leave the Uranium 238 nuclei almost unaffected. Anticipating the day when Uranium 235 will become scarce, several countries have built a different kind of reactor that contains no moderator. Such reactors carry out chain reactions with fast-moving neutrons and are thus called fast fission reactors. A fast fission reactor operates much like a controlled fission bomb and requires highly enriched uranium fuel as well. While a thermal fission reactor can get by with natural uranium or uranium that has been enriched to about 2–3% Uranium 235, a fast fission reactor needs 25–50% Uranium 235 fuel. That way, there are enough Uranium 235 nuclei around to maintain the chain reaction.
There is a side effect to operating a fast fission reactor. Many of the fast fission neutrons are captured by Uranium 238 nuclei, which then transform into Plutonium 239 nuclei. Thus the reactor produces plutonium as well as heat. For that reason, fast fission reactors are often called breeder reactors—they create new fissionable fuel. The Plutonium 239 can eventually replace the Uranium 235 as the principal fuel used in the reactor.
A thermal fission reactor makes some plutonium, which is usually allowed to fission in place, but a fast fission reactor makes a lot of it. Because plutonium can be used to make nuclear weapons, fast fission reactors are controversial. However, because they convert otherwise unusable Uranium 238 into a fissionable material, they use natural uranium far more efficiently than thermal fission reactors. One interesting complication of the unmoderated design is that fast fission reactors can't be water-cooled. If they were, the water would act as a moderator and slow the neutrons down. Instead, they are usually cooled with liquid sodium metal. The sodium nuclei of 23Na rarely interact with fast-moving neutrons, so they don't slow them down.
Fission Reactor Safety and Accidents
One of the greatest concerns with nuclear fission reactors is the control of radioactive waste. Anything that comes in contact with the reactor core or the neutrons it emits becomes somewhat radioactive. The fuel pellets themselves are quickly contaminated with all sorts of fission fragments that include radioactive isotopes of many familiar elements. Some of these radioactive isotopes dissolve in water or are gases, and all of them must be handled carefully.
The first line of defense against the escape of radioactivity is the large and sturdy containment vessel around the reactor. Because most of the radioactive materials remain in the reactor core itself or in the cooling fluid, they are trapped in the containment vessel. Whenever the nuclear fuel is removed for reprocessing, care is taken not to allow radioactive materials to escape.
The other great concern is the safe operation of the reactors themselves. Like any equipment, reactors experience failures of one type or another, and a safe reactor must not respond catastrophically to such failures. Toward that end, reactors have emergency cooling systems, pressure-relief valves, and many ways to shut down the reactor. For example, injecting a solution of sodium borate into the core cools it and stops any chain reactions. The boron nuclei in sodium borate absorb neutrons extremely well and are the main contents of most control rods. However, the best way to keep reactors safe is to design them so that they naturally stop their chain reactions when they overheat.
There have been five major reactor accidents since the dawn of the nuclear age. The first of these accidents began on October 7, 1957, during a maintenance procedure at Windscale Pile 1, one of Britain's two original plutonium production reactors. The reactor was cooled by air rather than water and had a graphite moderator. The reactor's intense radiation modified the graphite's crystalline structure so that it gradually accumulated large amounts of chemical potential energy. That potential energy had to be released periodically by heating the graphite to about 250 °C so that it recrystallized. During the accident, however, hot spots developed unexpectedly in the moderator and fuel rods, the metallic uranium in those rods ignited, and the burning reactor core distributed radioactive debris across the British countryside.
The second serious accident occurred at Three Mile Island on March 28, 1979. This pressurized water thermal fission reactor shut down appropriately when the pumps that circulated water in the power-generating loop stopped unexpectedly. Although this water loop wasn't directly connected to the reactor, it was important for removing heat from the reactor core. Even though the reactor shut down immediately, its neutron-absorbing control rods swiftly stopping its chain reaction, the radioactive nuclei created by recent fissions were still decaying and releasing energy. The core continued to release heat, and it eventually boiled the water in the cooling loop. This water escaped from the loop through a pressure-relief valve, and the top of the reactor core became exposed. With nothing to cool it, the core became so hot that it suffered permanent damage. Some of the water from the cooling loop found its way into an unsealed room, and the radioactive gases it contained were released into the atmosphere.
The third and most serious accident occurred at Chernobyl Reactor Number 4 on April 26, 1986. This water-cooled, graphite-moderated thermal fission reactor was a cross between a pressurized water reactor and a boiling water reactor. Cooling water flowed through the reactor at high pressure but didn't boil until it was ready to enter the steamgenerating turbines.
The accident began during a test of the emergency core-cooling system. To begin the test, the operators tried to reduce the reactor's fission rate. However, the core had accumulated many neutron-absorbing fission fragments, which made it incapable of sustaining a chain reaction at a reduced fission rate. The chain reaction virtually stopped. To get the chain reaction running again, the operators had to withdraw a large number of control rods. These control rods were motor-driven, and it would take about 20 seconds to put them back in again. The operators now initiated the test by shutting off the cooling water. That should have immediately shut down the reactor by inserting the control rods, but the operators had overridden the automatic controls because they didn't want to have to restart the reactor a second time. With nothing to cool it, the reactor core quickly overheated and the water inside it boiled. The water had been acting as a moderator along with the graphite. However, the reactor was overmoderated, meaning it had more moderator than it needed. Getting rid of the water actually helped the chain reaction because the water had been absorbing some of the neutrons. The fission rate began to increase.
The operators realized they were in trouble and began to shut down the reactor manually. However, the control rods moved into the core too slowly to make a difference. As water disappeared from the core, the core went “prompt critical.” The chain reaction no longer had to wait for neutrons from the decaying fission fragments because prompt neutrons from the Uranium 235 fissions were enough to sustain the chain reaction on their own. The reactor's fission rate skyrocketed, doubling many times each second. The fuel became white hot and melted its containers. Various chemical explosions blew open the containment vessel, and the graphite moderator caught fire.
The fire burned for 10 days before firefighters and pilots encased the wreckage in concrete. Many of these heroic people suffered fatal exposures to radiation. The burning core released all its gaseous radioactive isotopes and many others into the atmosphere, forcing the evacuation of more than 100,000 people and leading to thousands or perhaps tens of thousands of premature cancer deaths in the millions of people exposed to the radioactive debris. The accident site remains dangerously radioactive and will need careful tending for centuries.
Although not a true reactor accident, the September 30, 1999, disaster in Tokaimura, Japan, did involve a critical mass and a resulting chain reaction. At about 10:35 am, employees of the Conversion Test Facility of JCO Co., Ltd. Tokai Works were pouring a solution of uranyl nitrate into a precipitation tank. Destined for an experimental fast fission reactor, this uranium had been enriched to about 18.8% Uranium 235. Although the equipment and facilities were designed to prevent the assembly of a critical mass, the workers decided to save time by circumventing the safeguards and combining many small batches into one large one.
After they had poured six or seven batches of uranyl nitrate solution into the stainless steel tank through a sampling hole, the solution suddenly reached critical mass. There was about 16.6 kg of enriched uranium in the tank, and a sudden burst of radiation was released. The water temperature leapt upward, and the solution's resulting expansion dropped the mixture below critical mass. However, as heat flowed from the solution to the water-cooled jacket around the tank, the solution again approached critical mass. An episodic chain reaction continued in the tank for about 20 hours, until draining the cooling jacket of its neutron-reflecting water finally put an end to the critical mass.
The Fukushima Daiichi nuclear accident north of Tokyo, Japan is the fifth reactor accident, began on March 11, 2011, following the 9.0-magnitude Tohoku earthquake and the resulting tsunami. The Fukushima nuclear facility housed six separate boiling water reactors, but only reactors 1, 2, and 3 were operating at the time, and they shut down automatically after the earthquake. Even after stopping their chain reactions, however, those reactor cores continued to generate heat through the decay of their vast accumulations of radioactive nuclei, and they needed to be water-cooled to avoid overheating. Emergency generators began supplying power to maintain that cooling.
When the tsunami struck the Tohoku coast less than an hour after the earthquake, it inundated the nuclear facility, flooded its generators and switching equipment, and detached the entire area from the Japanese electric power grid. Reactor cooling soon ceased, and despite heroic efforts by emergency workers, it wasn't restored for days. By then, the reactors were damaged beyond repair, and the world's second worst nuclear accident was well underway.
Lacking adequate cooling, the cores of reactors 1, 2, and 3 gradually overheated and then melted. Explosions, pressure venting, and discharges of cooling water into the sea all contributed to an enormous release of radioactive nuclei into the environment. Even spent fuel in storage pools at the facility contributed to the overall accident because it also suffered overheating and damage when cooling was lost. The Fukushima Daiichi plant will never reopen and will remain a radioactive disaster area for years to come.
Nuclear Fusion Reactors
Nuclear fission reactors use a relatively rare fuel—uranium. Although Earth's supply of uranium is vast, most of that uranium is distributed broadly throughout Earth's crust. There are only so many deposits of high-grade uranium ores that are easily turned into pure uranium or uranium compounds. Fission reactors also produce all sorts of radioactive fission fragments that must be disposed of safely. There is still no comprehensive plan for the safekeeping of spent reactor fuels. These must be kept away from any contact with people and animals virtually forever. No one really knows how to store such dangerous materials for hundreds of thousands of years.
An alternative to nuclear fission is nuclear fusion. By joining hydrogen nuclei together, heavier nuclei can be constructed. The amount of energy released in such processes is enormous. Recall, however, that fusion is much harder to initiate than fission because it requires that at least two nuclei be brought extremely close together. These nuclei are both positively charged, and they repel one another fiercely. To make them approach one another closely enough to stick, we must heat the nuclei to temperatures of more than 100 million degrees Celsius.
The sun combines four hydrogen nuclei (1H) to form one nucleus of helium (4He), a very complicated and difficult nuclear fusion reaction. For fusion to occur on Earth, it must be done between the heavy isotopes of hydrogen: deuterium and tritium. These are the isotopes used in thermonuclear weapons. If a mixture of deuterium and tritium are mixed together and heated to about 100 million degrees Celsius, their nuclei will begin to fuse and release energy. The deuterium and tritium become helium and neutrons. In contrast to fission reactions, there are no radioactive fragments produced in fusion.
Tritium itself is radioactive, but it can easily be reprocessed into fuel and retained within the reactor system. The dangerous neutrons can be caught in a blanket of lithium metal, which then breaks into helium and tritium. It's convenient that new tritium is created by the reaction because tritium isn't naturally occurring and must be made using nuclear reactions. Thus, fusion holds up the promise of producing relatively little radioactive waste. If the neutrons that are released by fusion events are trapped by nuclei that don't become radioactive, then there will be no radioactive contamination of the fusion reactor either. This is easier said than done, but it's better than in a fission reactor.
Unfortunately, heating deuterium and tritium and holding them together long enough for fusion to occur isn't easy. There are two main techniques that are being tried: inertial confinement fusion and magnetic confinement fusion.
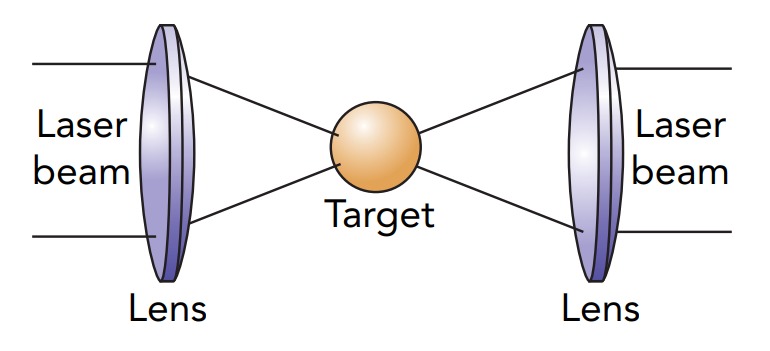
Inertial confinement fusion uses intense pulses of laser light to heat and compress a tiny sphere containing deuterium and tritium. The pulses of light last only a few trillionths of a second, but in that brief moment they vaporize and superheat the surface of the sphere. The surface explodes outward, pushing off the inner portions of the sphere. The sphere's core experiences huge inward forces as a result, and it implodes. As the core is compressed, its temperature rises to that needed to initiate fusion. In effect, it becomes a tiny thermonuclear bomb, with the laser pulses providing the starting heat.
To date, inertial confinement fusion experiments have observed fusion in a small fraction of the deuterium and tritium nuclei. The technique is called inertial confinement fusion because there is nothing holding or confining the ball of fuel. The laser beams crush it while it's in free fall, and its own inertia keeps it in place while fusion takes place. Unfortunately, the lasers and other technologies needed to carry out inertial confinement fusion are sufficiently complex and troublesome that this may never be viable as a source of energy. Nonetheless, these experiments provide important information on the behaviors of fusion materials at high temperatures and pressures.
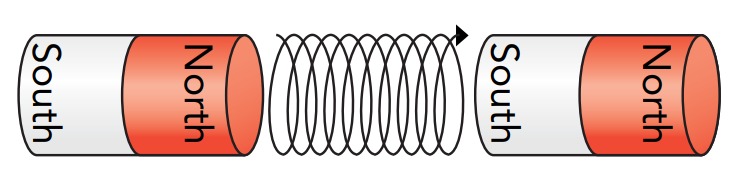
The other technique being developed to control fusion is magnetic confinement. When you heat hydrogen atoms hot enough, they move so quickly and hit one another so hard that their electrons are knocked off. Instead of a gas of atoms, you have a gas of free, positively charged nuclei and negatively charged electrons—a plasma. A plasma differs from a normal gas because it's affected by magnetic fields.
Because of the Lorentz force, charged particles that are moving in a magnetic field tend to circle around magnetic field lines. This cyclotron motion occurs, for example, in the magnetron of a microwave oven. If the magnetic field surrounding a charged particle is carefully shaped in just the right way, the charged particle will find itself trapped by the magnetic field. No matter what direction it heads, the charged particle will spiral around the magnetic field lines and will be unable to escape.
Magnetic confinement makes it possible to heat a plasma of deuterium and tritium to fantastic temperatures with electromagnetic waves. Since the heating is done relatively slowly, it's important to keep heat from leaving the plasma. Magnetic confinement prevents the plasma from touching the walls of the container, where it would quickly cool off.
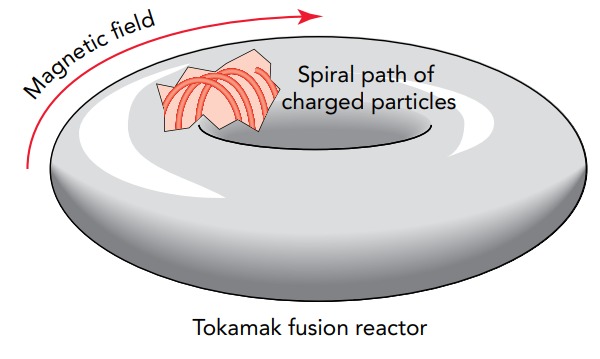
One of the most promising magnetic confinement schemes is the tokamak. The main magnetic field of the tokamak runs around in a circle to form a magnetic doughnut, or toroid. The magnetic field is formed inside a doughnut-shaped chamber by running an electric current through coils that are wrapped around the chamber. Plasma nuclei inside the chamber travel in spirals around the magnetic field lines and don't touch the chamber walls. They are confined inside the chamber and race around the doughnut endlessly. The nuclei can then be heated to the extremely high temperatures they need to collide and fuse.
Magnetic confinement fusion reactors have observed considerable amounts of fusion. They can briefly achieve scientific break-even, the point at which fusion is releasing enough energy to keep the plasma hot all by itself. However, much more development is needed to meet and exceed practical break-even, the point at which the entire machine produces more energy than it needs to operate.