Applications in Health Science
Some of the most important recent advances in health care have occurred at the border between medicine and physics. As scientists have refined their understanding of atomic and molecular structure and learned to control various forms of radiation, they have invented tools that are enormously valuable for diagnosing and treating illness and injury. The developments continue, with new applications of physics appearing in clinical settings almost every time you turn around. In this part, we'll examine two of the most significant examples of medical physics: the imaging techniques that are used to detect problems and the radiation therapies that are used to treat them.
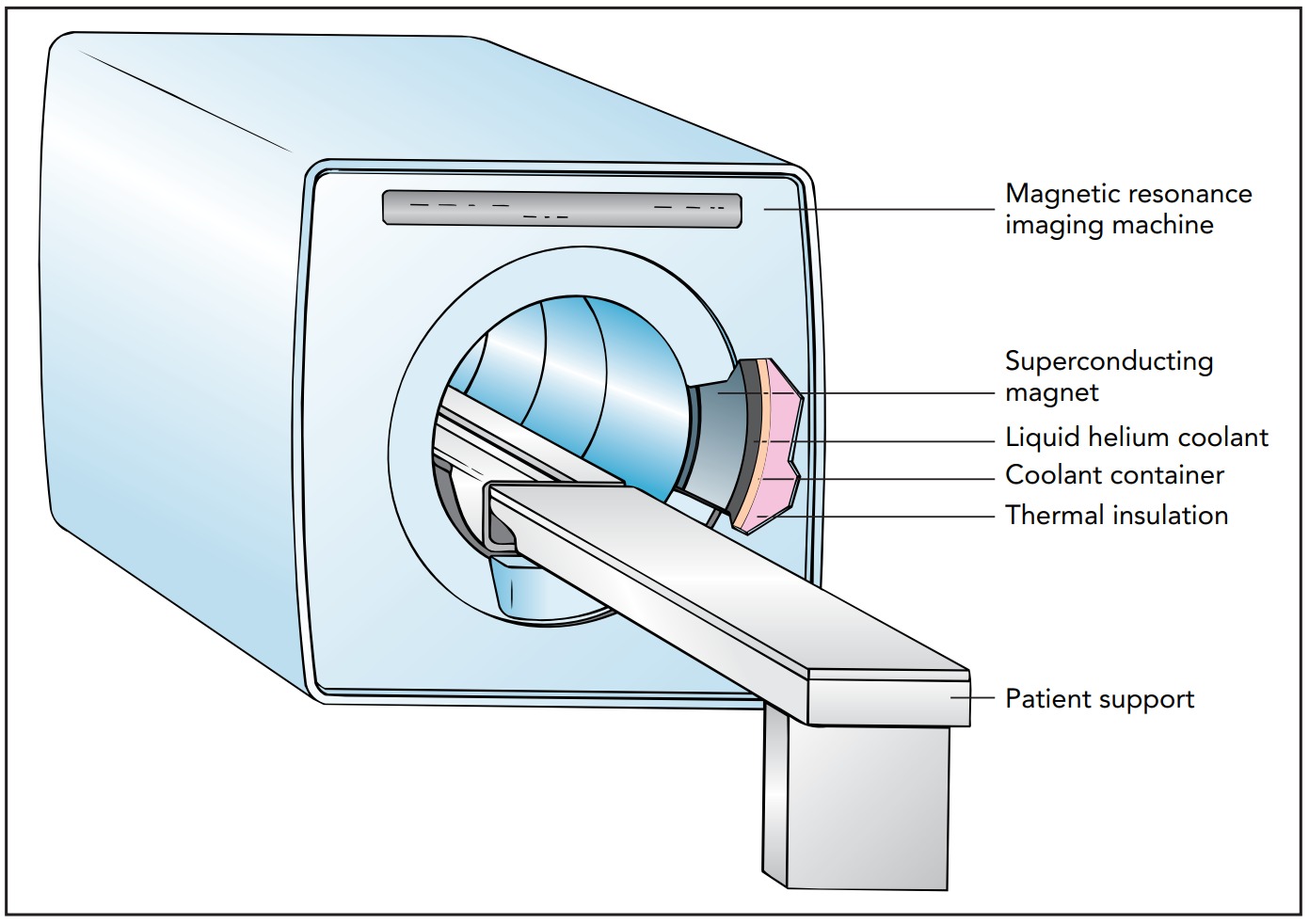
X-rays
Since their discovery in 1895, X-rays have played an important role in medical treatment. Their usefulness was obvious from the very evening they were discovered. It was November 8, and German physicist Wilhelm Conrad Roentgen (1845–1923) was experimenting with an electric discharge in a vacuum tube. He had covered the entire tube in black cardboard and was working in a darkened room. Some distance from the tube, a phosphored screen began to glow. Some kind of radiation was being released by the tube, passing through the cardboard and the air, and causing the screen to fluoresce. Roentgen put various objects in the way of the radiation, but they didn't block the flow. Finally, he put his hand in front of the screen and saw a shadowed image of his bones. He had discovered X-rays and their most famous application at the same time.
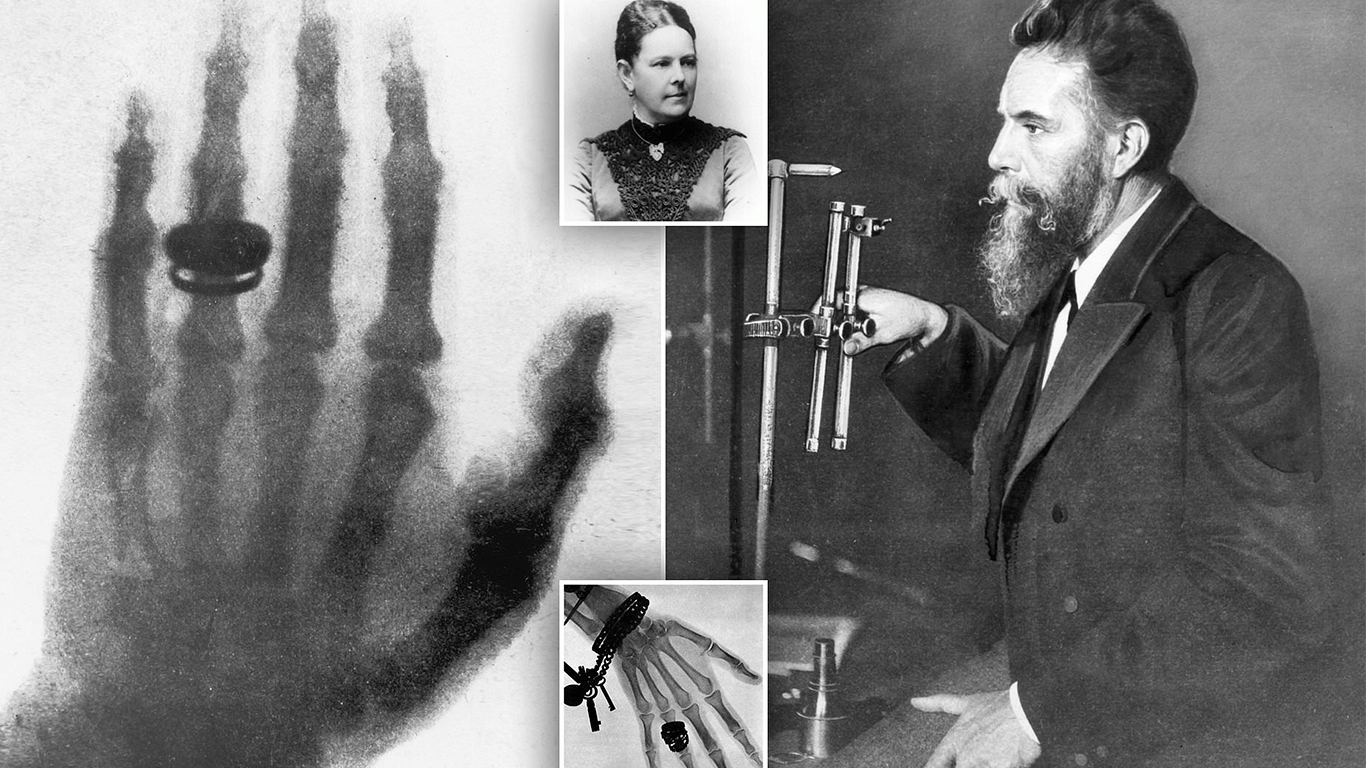
The first clinical use of X-rays was on January 13, 1896, when two British doctors used them to find a needle in a woman's hand. In no time, X-ray systems became common in hospitals as a marvelous new technique for diagnosis. However, this imaging capability was not without its side effects. Although the exposure itself was painless, overexposure to X-rays caused deep burns and wounds that took some time to appear. Evidently the X-rays were doing something much more subtle to the tissue than simply heating it.
X-rays are a form of electromagnetic radiation, as are radio waves, microwaves, and light. These different forms of electromagnetic radiation are distinguished from one another by their frequencies and wavelengths—while radio waves have low frequencies and long wavelengths, X-rays have extremely high frequencies and short wavelengths. They're also distinguished by their photon energies. Because of its low frequency, a radio wave photon carries little energy. A medium-frequency photon of blue or ultraviolet light carries enough energy to rearrange one bond in a molecule. But a high-frequency X-ray photon carries so much energy that it can break many bonds and rip molecules apart.
In a microwave oven, the microwave photons work together to heat and cook food. The amount of energy in each microwave photon is unimportant because they don't act alone. In radiation therapy, however, the X-ray photons are independent. Each one carries enough energy to damage any molecule that absorbs it. That's why X-ray burns involve little heat and appear long after the exposure—the molecular damage caused by X-rays takes time to kill cells
X-Rays generation
Medical X-ray sources work by crashing fast-moving electrons into heavy atoms. These collisions create X-rays via two different physical mechanisms: bremsstrahlung and X-ray fluorescence.
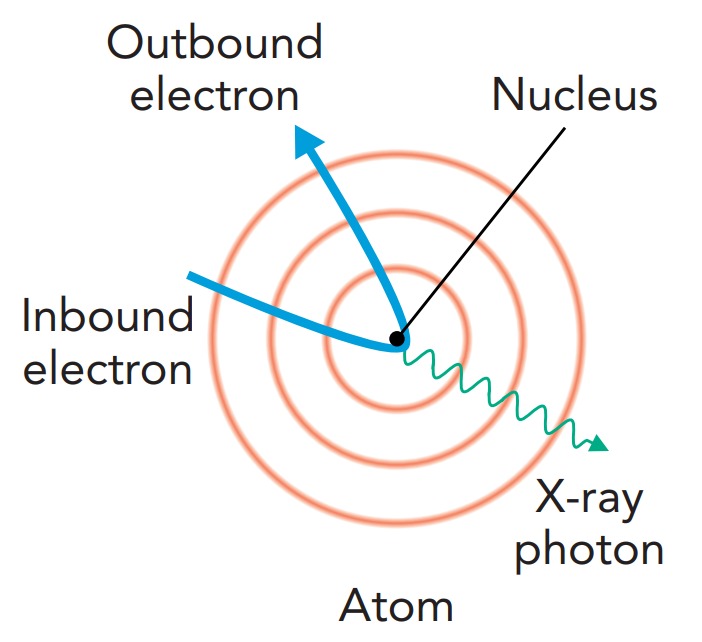
Bremsstrahlung occurs whenever a charged particle accelerates. This process is nothing really new, since we know that radio waves are emitted when a charged particle accelerates on an antenna. In a radio antenna, however, the electrons accelerate slowly and emit low-energy photons. Bremsstrahlung usually refers to cases in which a charged particle accelerates extremely rapidly and emits a very high-energy photon. In X-ray tube bremsstrahlung, a fast-moving electron arcs around a massive nucleus and accelerates so abruptly that it emits an X-ray photon. This photon carries away a substantial fraction of the electron's kinetic energy. The closer the electron comes to the nucleus, the more it accelerates and the more energy it gives to the X-ray photon. However, the electron is more likely to miss the nucleus by a large distance than to almost hit it, so bremsstrahlung is more likely to produce a lower-energy X-ray photon than a higher-energy one.
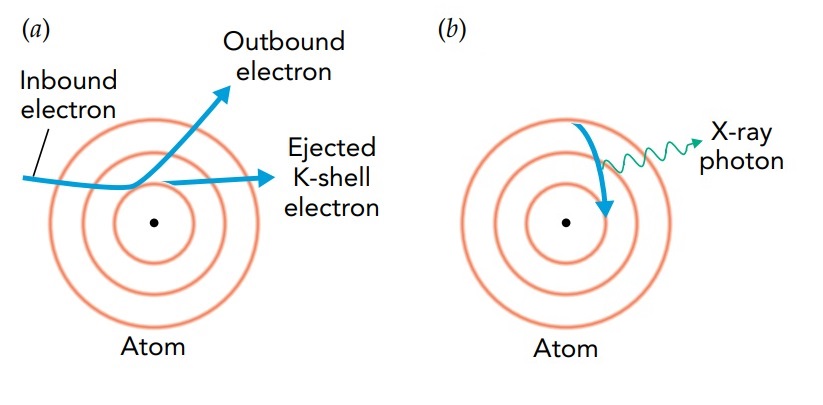
In X-ray fluorescence, the fast-moving electron collides with an inner electron in a heavy atom and knocks that electron completely out of the atom. The collision leaves the atom as a positive ion, with a vacant orbital close to its nucleus. An electron in that ion then undergoes a radiative transition, shifting from an outer orbital to this empty inner one and releasing an enormous amount of energy in the process. This energy emerges from the atom as an X-ray photon. Because this photon has an energy that's determined by the ion's orbital structure, it's called a characteristic X-ray.
To discuss the energies carried by X-ray photons, we'll use the energy unit — the electron volt (e V). Photons of visible light carry energies of between 1.6 electron volts (red light) and 3.0 electron volts (violet light). Because the ultraviolet photons in sunlight have energies of up to 7 electron volts, they are able to break chemical bonds and cause sunburns. X-ray photons have much larger energies than even ultraviolet photon.
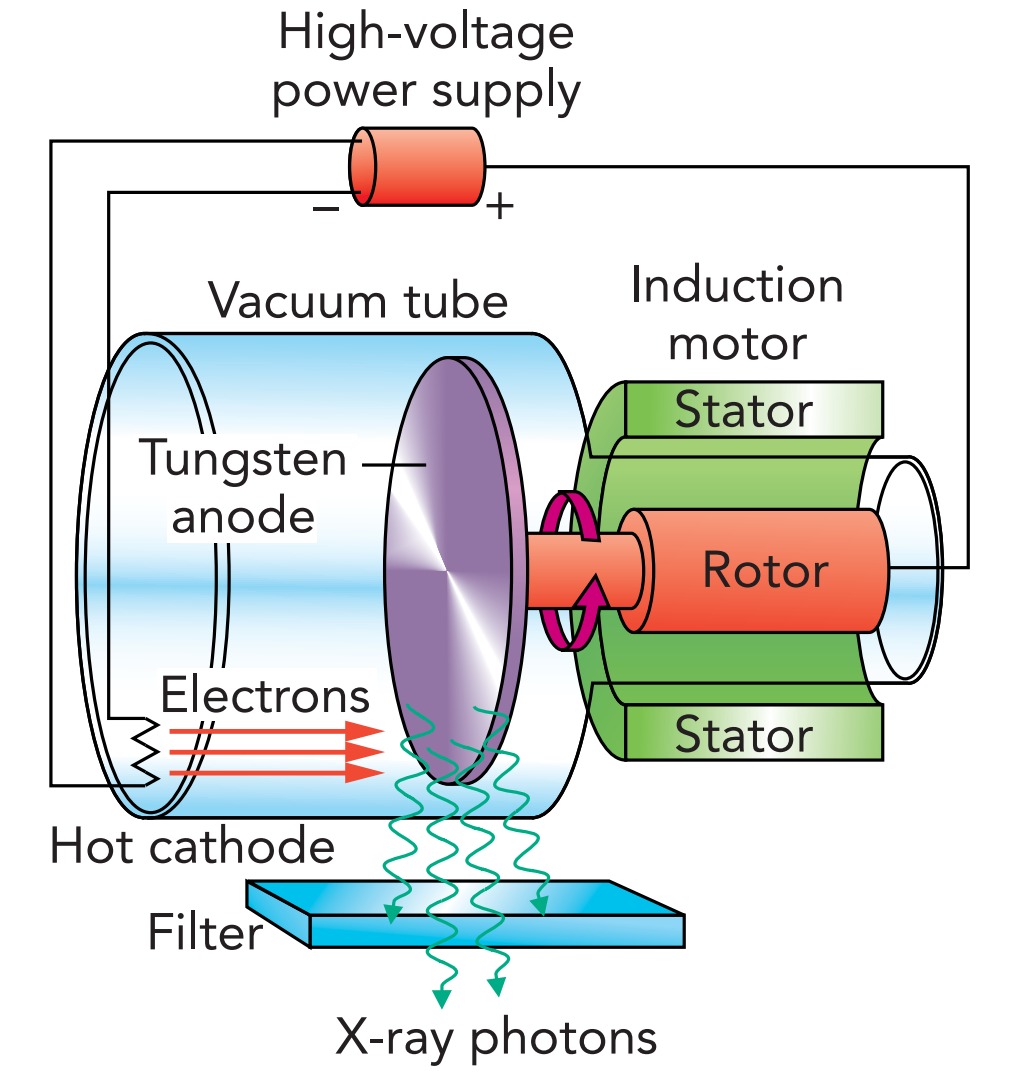
In a typical medical X-ray tube, electrons are emitted by a hot cathode and accelerate through vacuum toward a positively charged metal anode. The anode is a tungsten or molybdenum disk, spinning rapidly to keep it from melting. The energy of the electrons as they hit the anode is determined by the voltage difference across the tube. In a medical X-ray machine, that voltage difference is typically about 87,000 Volts, so each electron has about 87,000 e V of energy. Since an electron gives a good fraction of its energy to the X-ray photon it produces, the photons leaving the tube can carry up to 87,000 e V of energy. No wonder X-rays can damage tissue!
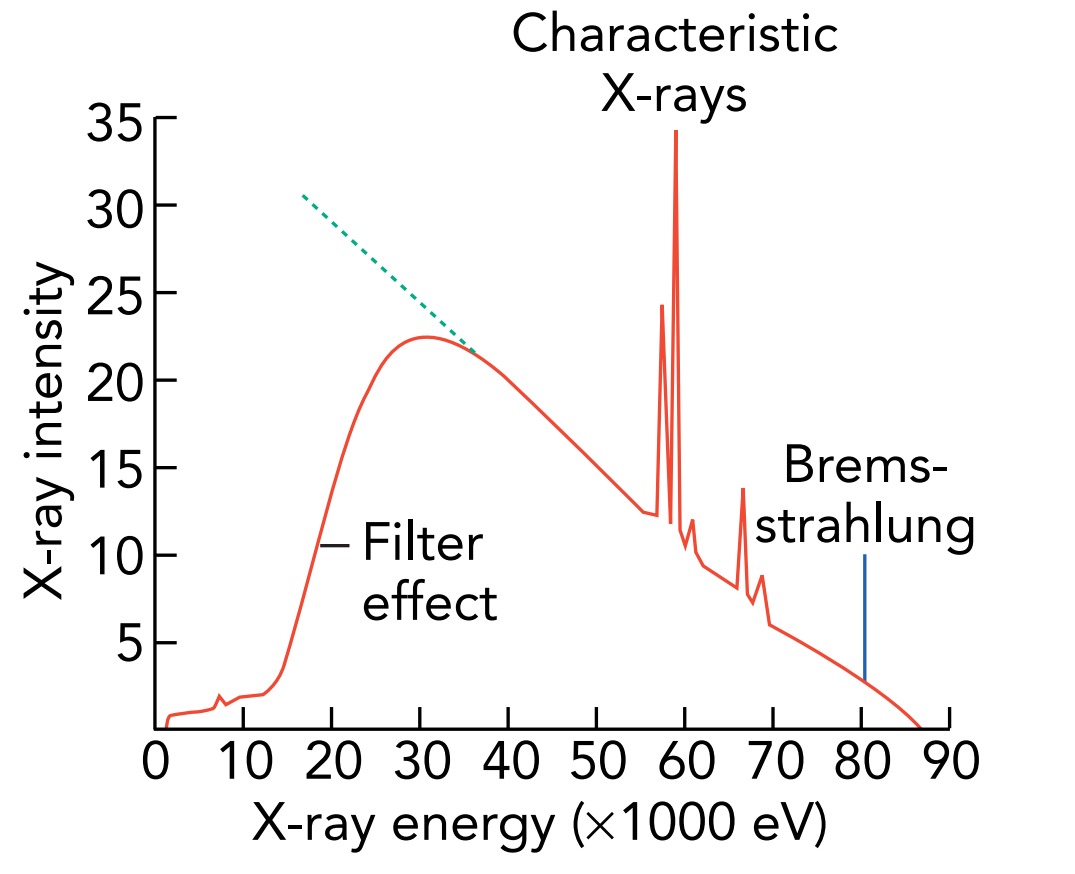
When the electrons collide with a target of heavy atoms, they emit both bremsstrahlung and characteristic X-rays. The characteristic X-rays have specific energies, so they appear as peaks in the overall X-ray spectrum. The bremsstrahlung X-rays have different energies but are most intense at lower energies. Because lower-energy X-ray photons injure skin and aren't useful for imaging or radiation therapy, medical X-ray machines use absorbing materials, such as aluminum, to filter them out
X-Ray Scanner
X-rays have two important uses in medicine: imaging and radiation therapy. In X-ray imaging, X-rays are sent through a patient's body to a sheet of film or an X-ray detector. Some of the X-rays manage to pass through tissue, but most of them are blocked by bone. The patient's bones form shadow images on the film behind them. In X-ray radiation therapy, the X-rays are again sent through a patient's body, but now their lethal interaction with diseased tissue is what's important.
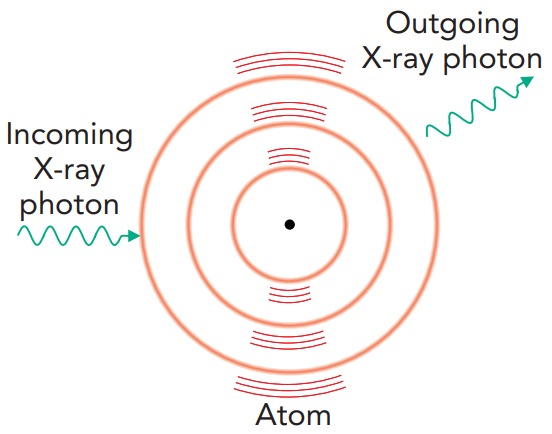
X-ray photons interact with tissue and bone through four major processes: elastic scattering, the photoelectric effect, Compton scattering, and electronpositron pair production. Elastic scattering is already familiar to us as the cause of the blue sky; an atom acts as an antenna for the passing electromagnetic wave, absorbing and reemitting it without keeping any of its energy. Because this process has almost no effect on the atom, elastic scattering isn't important in radiation therapy. However, it's a nuisance in X-ray imaging because it produces a hazy background; some of the X-rays passing through a patient bounce around like pinballs and arrive at the film from odd angles. To eliminate these bouncing X-ray photons, X-ray machines use filters to block X-rays that don't approach the film from the direction of the X-ray source.
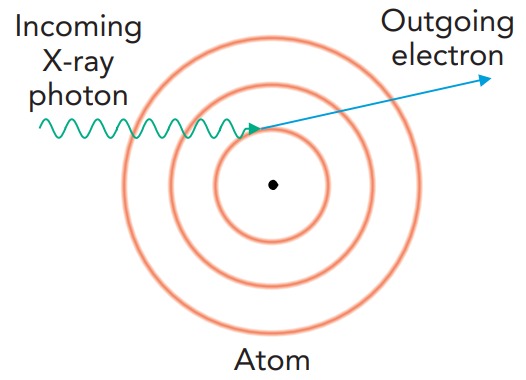
The photoelectric effect is what makes X-ray imaging possible. In this effect, a passing photon induces a radiative transition in an atom; one of the atom's electrons absorbs the photon and is tossed completely out of the atom. If the atom were using the X-ray photon to shift an electron from one orbital to another, that photon would have to have just the right amount of energy. However, because a free electron can have any amount of energy, the atom can absorb any X-ray photon that has enough energy to eject one of its electrons. Part of the photon's energy is used to remove the electron from the atom, and the rest is given to the emitted electron as kinetic energy.
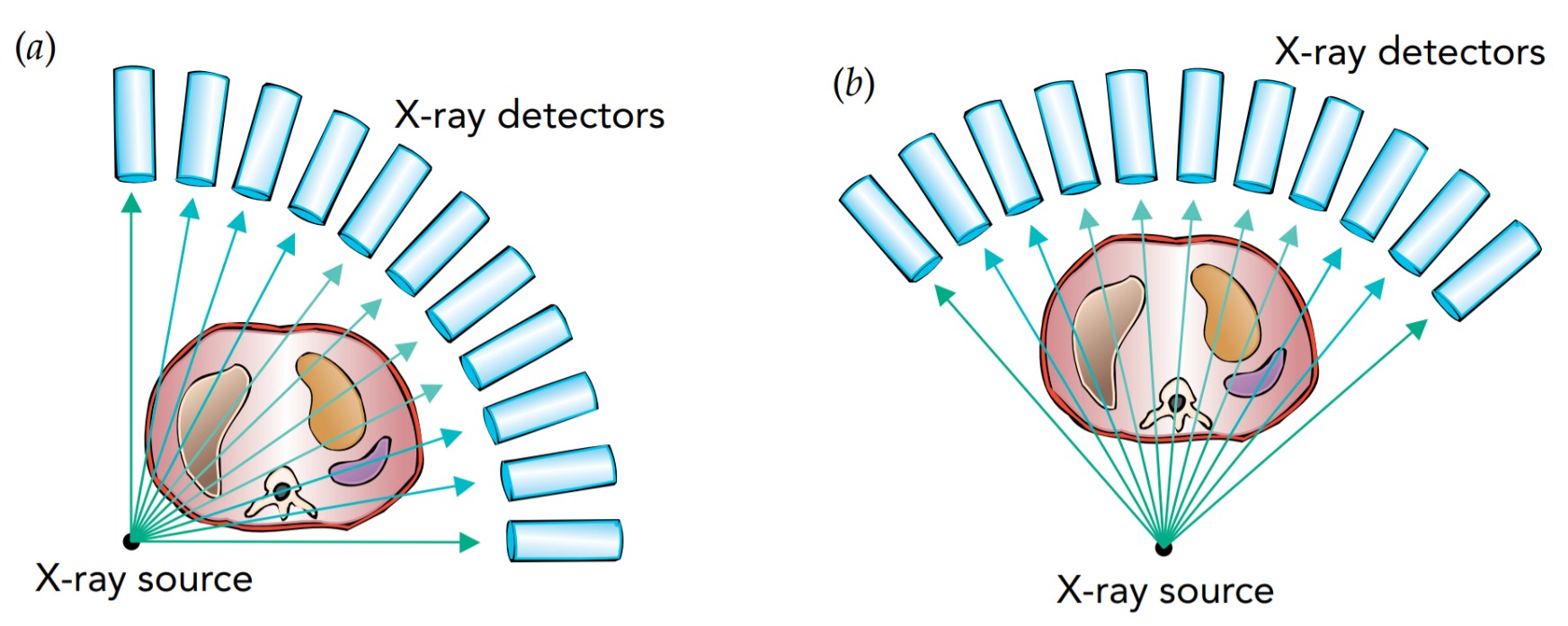
The likelihood of such a photoemission event decreases as the ejected electron's energy increases. This decreasing likelihood makes it difficult for a small atom to absorb an X-ray photon. All its electrons are relatively weakly bound, and the X-ray photon would give the ejected electron a large kinetic energy. Rather than emitting a high-energy electron, a small atom usually just ignores the passing X-ray photon.
In contrast, some of the electrons in a large atom are quite tightly bound and require most of the X-ray photon's energy to remove them. These electrons would depart with relatively little kinetic energy. Because the photoemission process is most likely when low-energy electrons are produced, a large atom is likely to absorb a passing X-ray. Thus, whereas the small atoms found in tissue (carbon, hydrogen, oxygen, and nitrogen) rarely absorb medical X-rays, the large atoms found in bone (calcium and phosphorus) absorb X-rays frequently. That's why bones cast clear shadows onto X-ray film. Tissue shadows are also visible, but they're less obvious.
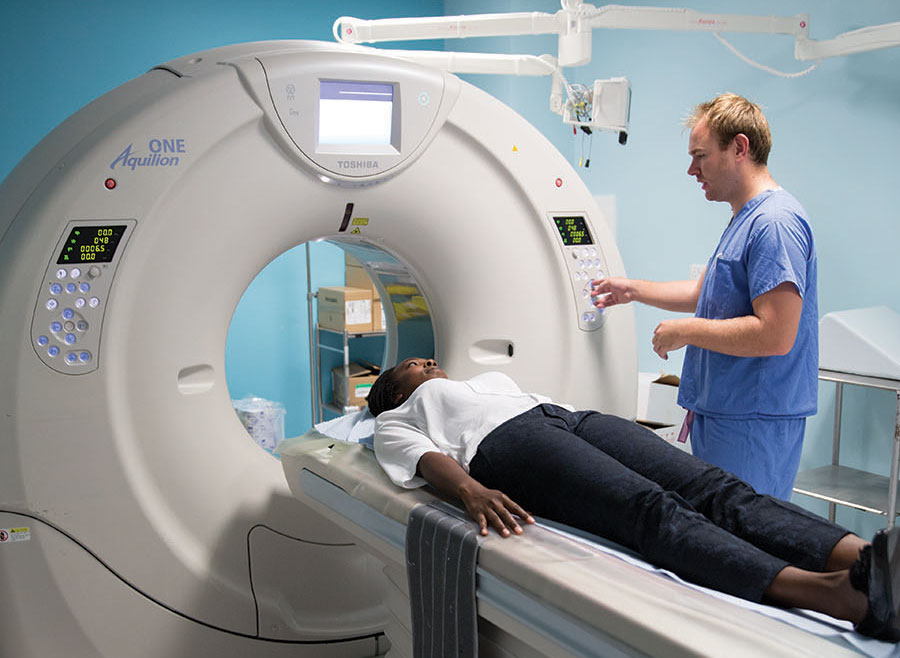
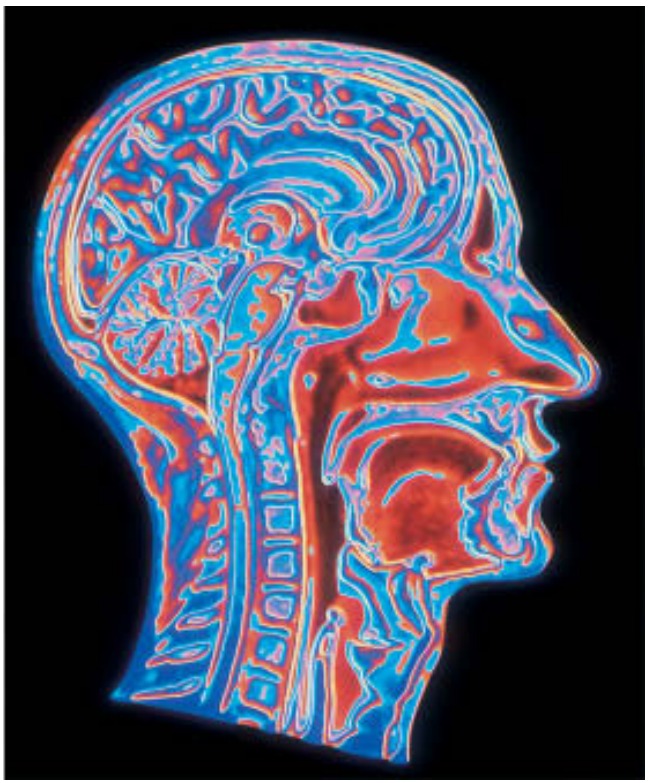
Although one shadow image of a patient's insides may help to diagnose a broken bone, more subtle problems may not be visible in a single X-ray image. For a better picture of what's going on inside the patient, the radiologist needs to see shadows from several different angles. Better yet, the radiologist can turn to a computed tomography (CT) scanner. This computerized device automatically forms X-ray shadow images from hundreds of different angles and positions and produces a detailed three-dimensional X-ray map of the patient's body.
The CT scanner works one slice of the patient's body at a time. It sends X-rays through this narrow slice from every possible angle, and determines where the bones and tissues are in that slice. The scanner then shifts the patient's body to work on the next slice.