Radiotherapy
Radiotherapy is a medical treatment that uses high-energy radiation to target and destroy cancer cells. It is one of the primary treatments for cancer, along with surgery and chemotherapy. Radiotherapy works by damaging the DNA within cancer cells, preventing them from dividing and growing. This ultimately leads to the death of the cancer cells or makes them unable to multiply further. Radiotherapy can be delivered externally or internally. The radiation is generated by a machine located outside the body and directed towards the tumor ine External Beam Radiotherapy. The patient lies on a treatment table, and the machine, often a linear accelerator, delivers precise doses of radiation to the tumor while minimizing exposure to surrounding healthy tissues. The radioactive sources are placed directly inside or next to the tumor in Internal Radiotherapy or also known as Brachytherapy. This can be done temporarily, where the sources are inserted for a specific amount of time and then removed, or permanently, where the sources are left in place. This method allows for a higher dose of radiation to be delivered directly to the tumor while reducing exposure to nearby healthy tissues.
Radiation therapy using X-Rays
Radiation therapy also uses X-rays, but not the ones used for medical imaging. Even though tissue absorbs fewer imaging photons than bone, most imaging photons are absorbed before they can pass through thick tissue. For example, only about 10% of the imaging photons make it through a patient's leg even when they miss the bone. That percentage is good enough for making an image, but it wouldn't do for radiation therapy because most imaging X-rays would be absorbed long before they reached a deep-seated tumor. Instead of killing the tumor, intense exposure to these X-rays would kill tissue near the patient's skin.
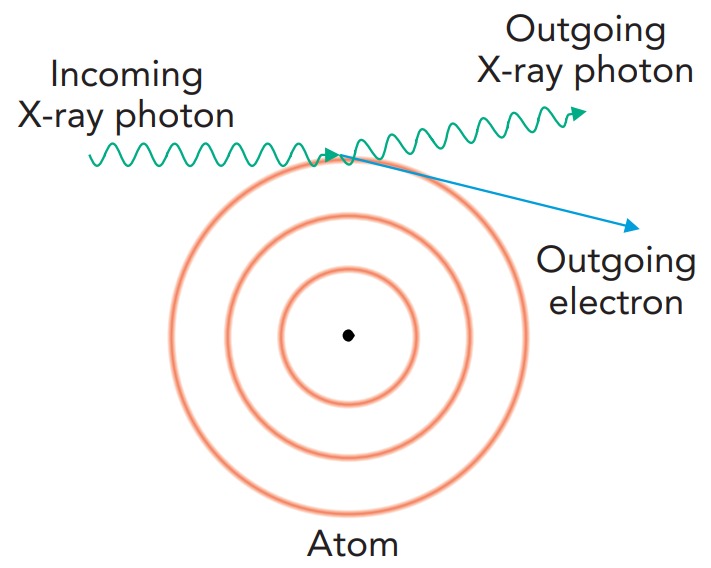
To attack malignant tissue deep beneath the skin, radiation therapy uses extremely high-energy photons. At photon energies near 1,000,000 electron Volts, the photoelectric effect becomes rare in tissue and bone, and the photons are much more likely to reach the tumor. Photons still deposit lethal energy in the tissue and tumor, but they do this through a new effect—Compton scattering.
Compton scattering occurs when an X-ray photon collides with a single electron so that the two particles bounce off one another. The X-ray photon knocks the electron right out of the atom. This process is different from the photoelectric effect because Compton scattering doesn't involve the atom as a whole and the photon is scattered or bounced rather than absorbed. The physics behind this effect resembles that of two billiard balls colliding, although it's complicated by the theory of relativity. The fact that it occurs at all is proof that a photon carries both energy and momentum and that these quantities are conserved when a particle of light collides with a particle of matter.
Compton scattering is crucial to radiation therapy. When a patient is exposed to 1,000,000-electron Volts photons, most of the photons pass right through, but a small fraction undergo Compton scattering and leave some of their energy behind. This energy kills cells and can be used to destroy a tumor. By approaching a tumor from many different angles through the patient's body, the treatment can minimize the injury to healthy tissue around the tumor while giving the tumor itself a fatal dose of radiation.
Compton scattering isn't the only effect that occurs when high-energy photons encounter matter. X-rays with slightly more than 1,022,000 electron Volts can do something remarkable when they pass through an atom; they can cause electron-positron pair production. A positron is the antimatter equivalent of an electron. Our universe is symmetrical in many ways, and one of its nearly perfect symmetries is the existence of antimatter. Almost every particle in nature has an antiparticle with the same mass but opposite characteristics. A positron, or antielectron, has the same mass as an electron, but it's positively charged. There are also antiprotons and antineutrons.
Antimatter doesn't occur naturally on Earth, but it can be created in high-energy collisions. When an energetic photon collides with the electric field of an atom, the photon can become an electron and a positron. In the previous section, we discussed matter becoming energy; pair production is an example of energy becoming matter. It takes about 511,000 electron Volts of energy to form an electron or a positron, so the photon must have at least 1,022,000 electron Volts to create one of each. Any extra energy goes into kinetic energy in the two particles.
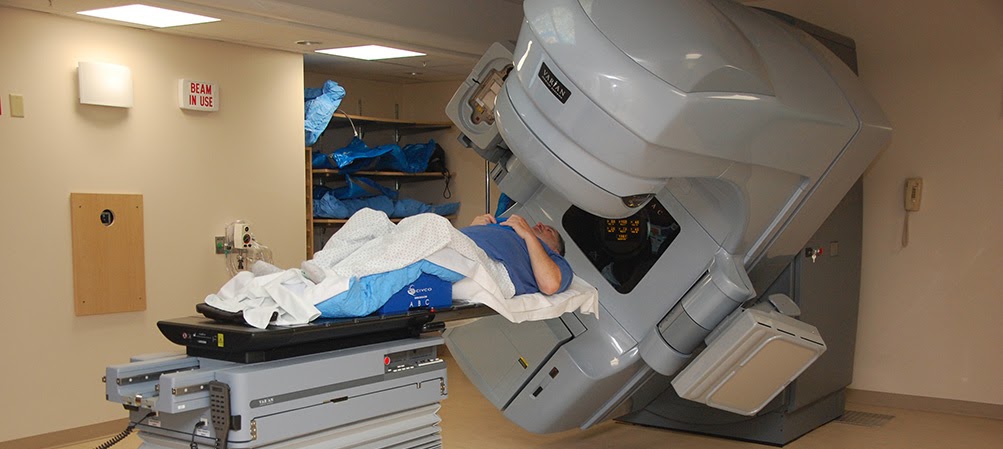
The positron doesn't last long in a patient. It soon collides with an electron and the two annihilate one another—the electron and positron disappear, and their mass becomes energy. They turn into photons with a total of at least 1,022,000 electron Volts. Thus energy became matter briefly and then turned back into energy. This exotic process is present in highenergy radiation therapy and becomes quite significant at photon energies above about 10,000,000 electron Volts. Not surprisingly, it also helps to kill tumors.
Radiation therapy using Gamma Rays
Producing very high-energy photons isn't quite as easy as producing those used in X-ray imaging. In principle, a power supply could create a huge voltage difference through an X-ray tube so that very high-energy electrons would crash into metal atoms and produce very high-energy photons. However, million-volt power supplies are complicated and dangerous, so other schemes are used instead.
One of the easiest ways to obtain very high-energy photons is through the decay of radioactive isotopes. The isotope most commonly used in radiation therapy is Cobalt 60. The nucleus of Cobalt 60 has too many neutrons and, like many neutron-rich nuclei, Cobalt 60 undergoes beta decay; that is, one of its neutrons breaks up into a proton, an electron, and a neutrino or more precisely, an antineutrino. Beginning with that beta decay, Cobalt 60 undergoes a series of transformations that produce two high-energy photons: one with 1,170,000 electron Volts and one with 1,330,000 electron Volts. These photons penetrate tissue well and are quite effective at killing tumors.
Although the process by which Cobalt 60 produces those two high-energy photons is complicated, beta decay itself shows that protons, electrons, and neutrons are not immutable and that there are other subatomic particles in our universe. Neutrons that are by themselves or in nuclei with too many neutrons are radioactive and experience beta decay. When that beta decay process occurs in a Cobalt 60 nucleus, the negatively charged electron and neutral neutrino quickly escape from the nucleus but the newly formed proton remains. The nucleus thus becomes Nickel 60.
The neutrino is a subatomic particle with no charge and little mass. Neutrinos aren't found in normal atoms. Although important in nuclear and particle physics, neutrinos are difficult to observe directly because they travel near the speed of light and hardly ever collide with anything. Without charge, they don't participate in electromagnetic forces and, unlike the electrically neutral neutron, they don't experience the nuclear force. They experience only gravity and the weak force, the last of the four fundamental forces known to exist in our universe. Because it's weak and occurs only between particles that are very close together, the weak force rarely makes itself apparent. One of the few occasions on which it plays an important role is in beta decay.
With almost no way to push or pull on another particle, a neutrino can easily pass right through the entire Earth. Neutrinos are detected occasionally, but only with the help of enormous detectors. That's why physicists first showed that neutrinos are emitted from decaying neutrons by measuring energy and momentum before and after the decay. The proton and electron produced by the decay don't have the same total energy and momentum as the neutron had before the decay. Something must have carried away the missing energy and momentum, and that something is the neutrino.
Once Cobalt 60 has turned into Nickel 60, the decay isn't quite over. The Nickel 60 nucleus that forms still has extra energy in it. Nuclei are complicated quantum physical systems, just as atoms are, and they have excited states, too. The Nickel 60 nucleus is in an excited state, and it must undergo two radiative transitions before it reaches the ground state. These radiative transitions produce very high-energy photons or gamma rays that are characteristic of the Nickel 60 nucleus: one with 1,170,000 electron Volts of energy and the other with 1,330,000 electron Volts. The gamma rays are what make Cobalt 60 radiation therapy possible.
Radiation therapy using Particle Accelerators
Electromagnetic radiation isn't the only form of radiation used to treat patients. Energetic particles such as electrons and protons are also used. Like tiny billiard balls, these fastmoving particles collide with the atoms inside tumors and knock them apart. As usual, this atomic and molecular damage tends to kill cells and destroy tumors.
However, obtaining extremely energetic subatomic particles isn't easy. High-voltage power supplies can be used to accelerate an electron or proton to about 500,000 electron Volts, but that isn't enough. When a charged particle enters tissue, it experiences strong electric forces and is easily deflected from its path. To make sure that it travels straight and true, all the way to a tumor, the particle must have an enormous energy. Giving each charged particle the millions or even billions of electron volts it needs for radiation therapy takes a particle accelerator.
Particle accelerators use resonant cavities, the microwave-frequency tank circuits. Each of these metal chambers acts simultaneously as a capacitor and an inductor and thus has a natural resonance for sloshing charge. In the resonant cavities of a particle accelerator, this sloshing charge creates huge electric fields that change with time. Those electric fields push charged particles through space until they reach incredible energies.
One important type of particle accelerator is the linear accelerator. In this device, the electric fields in a series of resonant cavities push charged particles forward in a straight line. Each of these cavities has charge sloshing back and forth rhythmically on its wall. When a small packet of charged particles enters the first cavity through a hole, it's suddenly pushed forward by the strong electric field inside that cavity. The packet accelerates forward and leaves the first resonant cavity with more kinetic energy than it had when it arrived—the electric field in that cavity has done work on the packet.
If the fields in the cavities were constant, the electric field in the second cavity would slow the packet down. You can see that the electric field in the second cavity points in the wrong direction. By the time the packet reaches the second cavity, the charge sloshing in its walls has reversed and so has the electric field. The packet is again pushed forward, and it emerges from the second cavity with still more kinetic energy.
Each resonant cavity in this series adds energy to the packet, so that a long string of cavities can give each of the packet's charged particles millions or even billions of electron volts. This energy comes from microwave generators that cause charge to slosh in the accelerator's resonant cavities. The linear accelerator then has only to inject charged particles into the first cavity, using equipment resembling the insides of a television picture tube, and those charged particles will come flying out of the last cavity with incredible energies.
This acceleration technique, however, has a few complications. Most important, each cavity must reverse its electric field at just the right moment to keep the packet accelerating forward. For simplicity of operation, all the cavities have the same resonant frequency and reverse their electric fields simultaneously. Since the packet spends the same amount of time in each cavity and since it speeds up as it goes from one cavity to the next, each cavity must be longer than the previous one.
As the packet approaches the speed of light, something strange happens. The packet's energy continues to increase as it goes through the cavities, but its speed stops increasing very much. This effect is a consequence of special relativity, the rules governing motion at speeds comparable to the speed of light. As a further consequence of relativity, the packet can approach the speed of light but can't actually reach it. Although each charged particle's kinetic energy can become extraordinarily large, its speed is limited by the speed of light.
Because the packet's speed stops increasing significantly after it has gone through the first few cavities of the linear accelerator, the lengths of the remaining cavities can be constant. Only the first few cavities have to be specially designed to account for the packet's increasing speed inside them. The charged particles emerge from the accelerator traveling at almost the speed of light. They pass through a thin metal window that keeps air out of the accelerator and enter the patient's body. They have so much energy that they can penetrate deep into tissue before coming to a stop.