Discharge Lamp
Energy efficiency is crucial to modern lighting. Incandescent lamps provide pleasant, warm illumination, but most of the power they consume is wasted as invisible infrared light. Fluorescent and other gas discharge lamps produce far more visible light with the same amount of electric power and now dominate office, industrial, and street lighting.
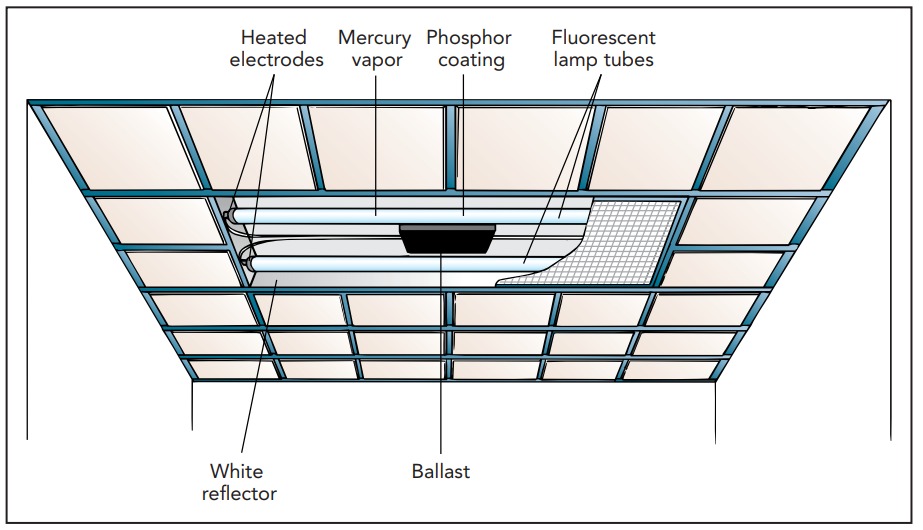
Before examining discharge lamps, let's look at how our eyes recognize color. It might seem that they actually measure the wavelengths of light, but that's not the case. Instead, our retinas contain three groups of light-sensing cone cells that respond to three different ranges of wavelengths. One group of cone cells responds to light near 600 nanometer and lets us see red, another responds to light near 550 nanometer and lets us see green, and a third responds to light near 450 nanometer and lets us see blue. These cone cells are most abundant at the center of our vision. Our retinas also contain rod cells, which are more light-sensitive than cone cells, but rod cells can't distinguish color. They are most abundant in our peripheral vision and provide us with night vision.
Having only three types of color-sensing cells doesn't limit us to seeing just three colors. We perceive other colors whenever two or more types of cone cells are stimulated at once. Each type of cell reports the amount of light it detects, and our brains interpret the overall response as a particular color.
Although light of a certain wavelength will stimulate all three types of cone cells simultaneously, the cells don't respond equally. If the wavelength is 680 nanometer, the red cone cells will respond much more strongly than the green or blue cells. Because of this strong red response, we see the light as red.
Other wavelengths of light stimulate the three types of cells somewhat more evenly. Light with a wavelength of 580 nanometer is in between red and green light. Both the red-sensitive and the green-sensitive cone cells respond about equally to this light, and we see it as yellow.
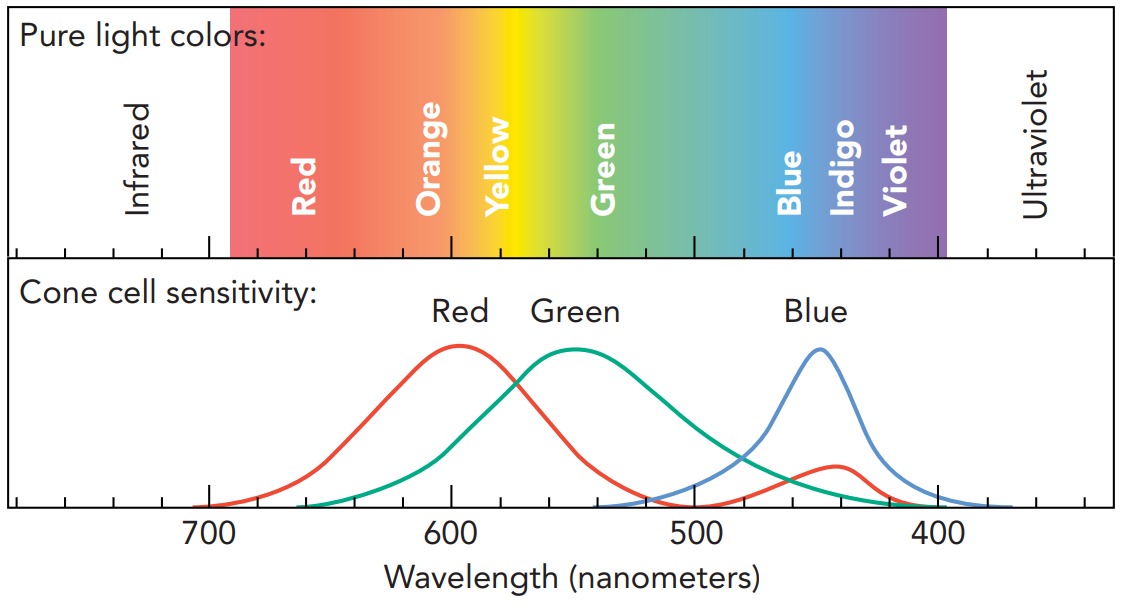
We also see yellow when looking at an equal mixture of 640-nanometer (red) light and 525-nanometer (green) light . The 640-nanometer light stimulates the red-sensitive cone cells and the 525-nanometer light stimulates the green-sensitive ones. Even though there is no 580-nanometer light entering our eyes, we see the same yellow color as before.
In fact, mixtures of red, green, and blue light can make us see virtually any color. For that reason, these three are called the primary colors of light or the primary additive colors. Color televisions and computer screens use tiny sources of red, green, and blue light to produce their full-color images.
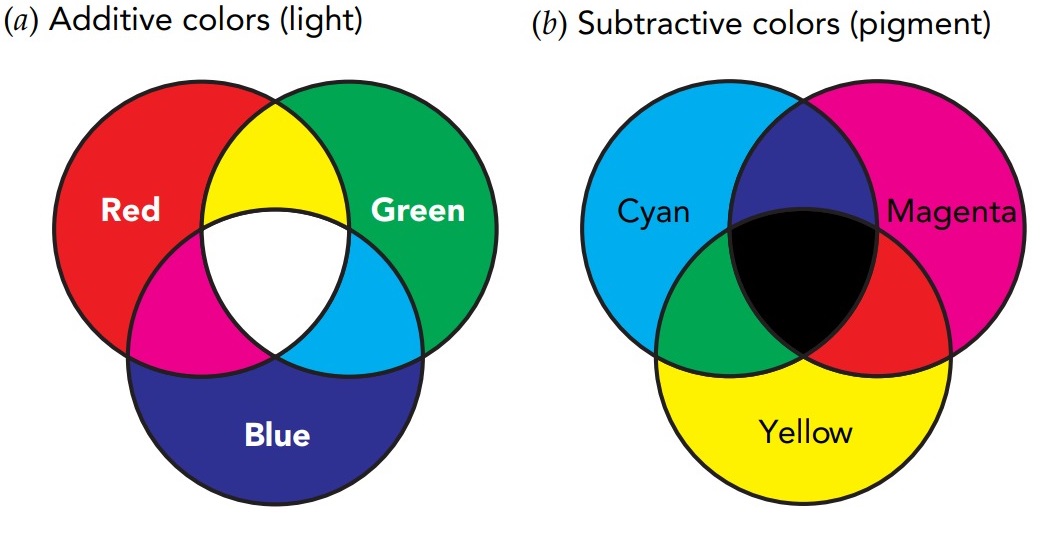
Although the idea of mixing primary colors also applies to paints, inks, and pigments, the palette is different. The primary colors of pigment or the primary subtractive colors are cyan, magenta, and yellow. When you apply one of these primary pigments to a white surface, it absorbs or subtracts one of the primary colors of light from the surface's reflection. Cyan subtracts the reflection of red, magenta subtracts the reflection of green, and yellow subtracts the reflection of blue. Color printers, photographs, magazines, and books use tiny patches of cyan, magenta, and yellow pigments to produce their full-color images.
Gas Discharges
When all three of our color-sensing cells respond about equally, we see white light. That's because our vision evolved under a single incandescent light source-the sun. Sunlight stimulates the red-, green-, and blue-sensitive cells in our eyes about evenly, so any other source of “white light” must do the same.
Most of the twentieth century's white-light illumination came from incandescent lightbulbs, but that won't be true for the twenty-first century. Although an incandescent lightbulb makes a good attempt at producing white light, it suffers from two serious drawbacks. First, because its filament can't reach the temperature of the sun's surface (or 5800 K), its blackbody spectrum contains too little blue light and appears redder than sunlight. Second, because most of its electromagnetic radiation is invisible infrared light, it doesn't use energy efficiently to produce visible light. It produces only about 15 lumens per watt, where the lumen is a standard measure of usable illumination.
Fortunately, modern science has given us some alternative sources of light, sources that don't use heat and thermal radiation to produce their light. Among these sources are gas discharge lamps, which emit light when electric currents pass through their gases. Some discharge lamps are colored, and others do excellent jobs of producing white light. Also, many of them are far more energy efficient at producing visible light than are incandescent lightbulbs.
To get an understanding of gas discharges and the lights they emit, let's start with one of the simplest examples, a neon sign tube. Although neon's rich red glow isn't suitable for lighting and isn't particularly energy efficient, it's great for signs and also relatively easy to understand.
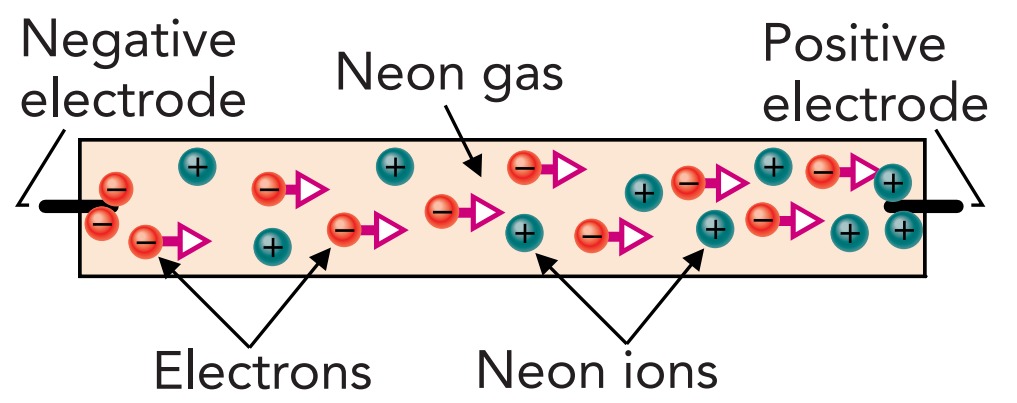
A neon sign tube is a sealed glass tube that contains pure neon gas at a density less than 1% that of the atmosphere outside the tube. It has metal electrodes at each end so that electric current can enter the gas through one electrode and leave through the other. Of course, gases are normally electrical insulators, and neon is no exception. To transform the tube's neon into a conductor, a large voltage difference is applied between its two electrodes. A gas breaks down when exposed to large voltage gradients; its few naturally occurring ions initiate a cascade of ionizing collisions that quickly fill the gas with charged particles and render it conducting.
Whereas ordinary air breaks down at a voltage gradient of about 30,000 Volts per cm, low-density neon breaks down at a much lower voltage gradient. That's because in a low-density gas, charged particles can travel farther and accumulate more kinetic energy before each collision. When about 10,000 Voltsis applied between the electrodes of a neon sign tube, the gas breaks down and begins to emit its familiar red glow.
The lamp is then experiencing a discharge; that is, current is flowing through the neon gas. This current consists mostly of electrons, flowing from the tube's negatively charged electrode to its positively charged electrode. Although these electrons collide frequently with neon atoms, they have so little mass that they usually just bounce off the atoms without losing much energy. Like Ping-Pong balls rebounding from elephants, the electrons do most of the bouncing and then continue on their way.
Every so often, however, an electron will collide with a neon atom and something different will happen; the neon atom will rearrange internally and absorb part of the electron's kinetic energy. The electron will rebound with less energy than it had before, and the neon atom will go on to emit light, probably red light. To understand why that light is probably red, however, we'll have to look at quantum physics and the structure of the neon atom.
Particles and Waves
As quantum physics gradually revealed itself to the scientists of the early twentieth century, they found the experience both exhilarating and disorienting. Prior to that era, the physical world seemed to divide neatly into particles and waves: scientists viewed an electron only as a particle and light only as a wave. However, one of the most basic observations of quantum physics, and the one most relevant to our present topic, is that everything has both particle and wave characteristics. Put simply, everything begins and ends as a particle, but travels as a wave.
For an electron, the quantum surprise is that it travels as a wave. For light, the quantum surprise is that it is emitted and absorbed as a particle. Called the wave-particle duality, this observation that everything in nature has both particle and wave characteristics has left few areas of physics unaffected. But although quantum physics is now a basic and essential part of nearly all modern physics research, its effects are subtle and often nonintuitive. They are most apparent in the microscopic world and are visible to us only indirectly. No wonder they seem so strange.
Electron Standing Waves in Atoms: Orbitals
According to the wave-particle duality, electrons have both particle and wave characteristics. Like all objects in our universe, electrons travel as waves when they move from place to place, and it's only when you go looking for them that you find them as particles at particular locations. When they reside in atoms and you leave them alone, electrons are best understood as waves.
In a nonquantum world, an electron would exist only as a particle and would be able to move at any speed along any path, even in an atom. However, ours is a quantum world and the electron does not travel as a particle at all; it travels as a wave. The electron is therefore constrained by the rules that govern waves. Like the confined mechanical waves on a violin string, drumhead, or basin of water, the confined electron waves in an atom have limited possibilities.
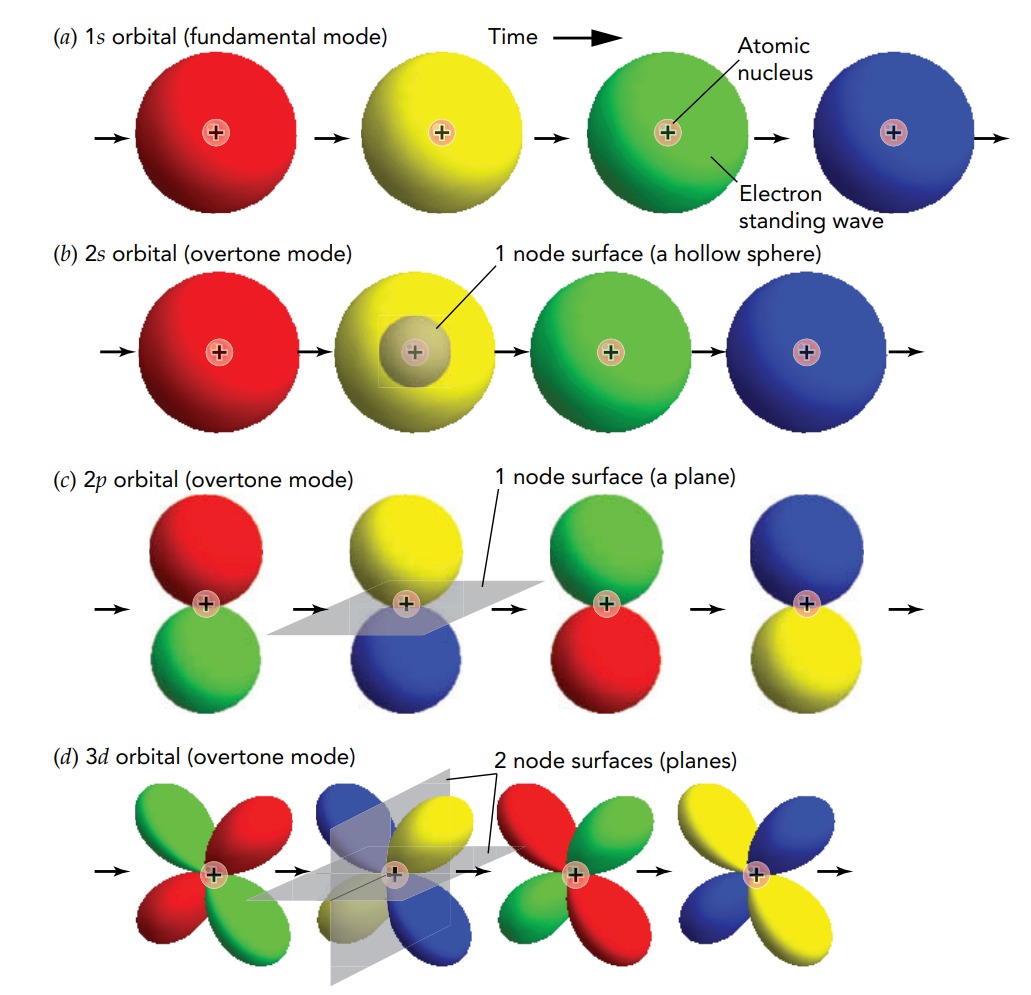
The most basic mechanical waves on a limited object are all standing waves-waves that effectively oscillate in place. This rule also applies to quantum waves in limited objects. An atom is a limited object, and the electrons in an atom are best understood as standing waves in that atom. Each electron wave extends across the atom and has such wave characteristics as wavelength and frequency.
Unlike a vibrating string or drumhead, however, the electron is a three-dimensional wave and its oscillation is internal. Instead of vibrating back and forth, each point of the wave cycles invisibly around a quantum phase. Since that quantum phase is rather mysterious, you can think of an electron standing wave as a fuzzy cloud in which each point cycles endlessly through a series of colors: red, yellow, green, blue, red, yellow, and so on. All those points cycle at the same quantum frequency, but they're not necessarily the same color at the same time. When the electron is in a three-dimensional standing wave, the electron cloud's spatial structure is constant as the wave oscillates internally and the electron exhibits no motion at all.
This wave character has profound effects on the electronic structure of atoms. Most significantly, it limits what electrons can do in those atoms. A violin string's one-dimensional standing waves consist only of its fundamental mode and its harmonic modes, and a drumhead's two-dimensional standing waves consist only of its fundamental mode and overtones. Similarly, an electron's three-dimensional standing waves in an atom consist only of a fundamental mode and overtone modes. Although there are a great many overtone modes available to the electron, their possibilities are nonetheless limited.
In short, In an atom, an electron normally exists in a three-dimensional quantum standing wave or orbital. As time passes, that wave undergoes an internal oscillation, depicted here as the color sequence red, yellow, green, blue, and so on. The 1s orbital is the electron's fundamental mode in an atom. It has the lowest energy and slowest quantum frequency of all the atom's electron modes.
The 2s orbital is an overtone mode with somewhat more energy and a faster quantum frequency than the 1s orbital. It has one node surface-a hollow sphere. One of three possible 2p orbitals; these are overtone modes that have energies and quantum frequencies similar to those of the 2s orbital. Each 2p orbital has one node surface-a plane. One of five possible 3d orbitals; these are overtone modes with still greater energies and faster quantum frequencies. Each 3d orbital has two node surfaces-both are planes.
The electron standing waves in atoms are known as orbitals-a nod to the orbits that electrons would make around atomic nuclei if this were a nonquantum world. Electrons in solids are less focused on specific atomic nuclei, so the standing waves in solids are instead called levels-a recognition that each standing wave has an amount, or level, of energy associated with it. Each solid's limited level choices determine whether it's a conductor, an insulator, or a semiconductor- the basis for modern electronics. An atom's limited orbital choices determine the colors of light it can emit or absorb and therefore the characteristics of most discharge lamps.
Atoms
Another remarkable observation of quantum physics is that every indistinguishable electron must have its own orbital or level, its own unique quantum wave. This law is called the Pauli exclusion principle, after its discoverer, Wolfgang Pauli 4 . The principle applies to a whole class of subatomic particles, the Fermi particles, that includes all the basic constituents of matter: electrons, protons, and neutrons. For reasons that lie deep in what is known as quantum field theory, two indistinguishable Fermi particles can never be in the same quantum wave.
However, a peculiar property of electrons allows two of them to share an orbital or level. Electrons have two possible internal states, usually called spin-up and spin-down. Because a spin-up electron is distinguishable from a spin-down electron, one spin-up electron and one spin-down electron can share a single orbital or level. However, two electrons is the absolute maximum allowed by quantum physics and the Pauli exclusion principle.
Despite being a wave, an electron in an orbital has a specific total energy-the sum of its kinetic and potential energies. That energy, which depends on the shape and structure of the electron wave, also determines the wave's oscillation frequency. According to quantum physics, the electron's total energy and the oscillation frequency of its wave are exactly proportional to one another; a low-energy electron oscillates slowly, while a high-energy electron oscillates quickly. Each orbital, each quantum standing wave in an atom, has a specific frequency and energy. The electron's fundamental mode (the 1s orbital) has the lowest frequency and energy, while the overtone modes have progressively higher frequencies and energies.
Although these orbitals are three-dimensional standing waves, they resemble the two-dimensional standing waves of the drumhead. Like the drumhead's vibrational modes, the orbitals are distinguished from one another by their patterns of nodes-surfaces along which the electron wave has no amplitude. An electron's fundamental mode has no nodes and has the lowest quantum frequency. Each of the overtone modes, however, has at least one node. In general, the more nodes an orbital has, the greater its quantum frequency.
Physicists have come to view these standing waves as abstract placeholders, independent of the electrons that may or may not exhibit them at a given moment. The orbitals are then analogous to seats in an stadium, each of which may or may not be occupied by electrons. Instead of saying that the electron exhibits a particular standing wave or orbital, we often say that the orbital is occupied by an electron.
At sufficiently low temperature, electrons occupy those orbitals that have the least energy and fill the atom's orbitals from lowest energy on up. In accordance with the Pauli exclusion principle, each orbital accommodates two electrons, one spin-up and one spindown, until all the atom's electrons have been placed. The chemical nature of a particular atom, and its location in the periodic table of the elements, is determined by how many electrons it has and how those electrons fill the available orbitals. Atoms are electrically neutral, so the number of negatively charged electrons in an atom is the same as the number of positively charged protons in its nucleus, its atomic number.
There are patterns to orbitals; orbitals may share the same number of nodes or be rotated versions of one another. These patterns among orbitals give rise to the shells, that is, groups of orbitals that have similar energies and tend to fill with electrons at about the same time. Although the orbital patterns and shells are complicated by the fact that charged electrons influence one another and distort one another's standing waves, many atomic properties are determined simply by which orbitals are occupied by an atom's electrons.
The atomic orbitals are identified primarily by an integer and a letter, both of which relate to their node patterns. The integer (1, 2, 3,…) is one more than the number of node surfaces in the orbital (0, 1, 2,…) and the letter (s, p, d, f, g, h,…) indicates how many of those node surfaces pass through the atom's center (0, 1, 2, 3, 4, 5,…). Although s orbitals appear one at a time, p orbitals appear in groups of three, d orbitals in groups of five, f orbitals in groups of seven, and so on.
Neon's Red Glow
A neon atom has 10 electrons, so it takes 5 orbitals to accommodate them. The first two electrons go into the 1s orbital, the fundamental mode with zero nodes. Filling the 1s orbital completes the first major shell. The next two electrons go into the 2s orbital, which has one node surface, a hollow sphere. Finally, neon's last six electrons go into the three 2p orbitals, each of which has one node surface, a plane passing through the atom's center. Since filling the 2s and 2p orbitals completes the second major shell, the neon atom (N e) is chemically inert. That is why neon exists as a gas of individual atoms!
An arrangement of occupied orbitals is called a state, and the state we have just described is neon's ground state, the state with the lowest possible total energy. The neon atom has other states available, but they all involve additional energy. That's where the discharge enters the picture. When a charged particle collides with a ground-state neon atom, there's a chance that the collision will knock an electron out of its usual orbital into one of the empty orbitals. The neon atom will then be in an excited state-it will have extra energy.
Suppose, for example, that a collision has just shifted one of the neon atom's electrons from a 2p orbital to a 3p orbital. The atom will quickly undergo a series of stateto-state transitions that eventually return it to its ground state. With each transition, the atom will drop to a lower energy state and emit a photon, a particle, or quanta, of light. The photon carries away the energy released in transitioning from the higher-energy state to the lower-energy state. In all likelihood, one of those transitions will shift the electron from its 3p orbital to the 3s orbital and produce a photon of red light, the red of a neon sign!
As long as the electron remains in the 3p orbital-a particular standing wave-it can't emit an electromagnetic wave. That standing wave has a quantum oscillation; however, the oscillation is internal to the electron and neither the electron wave nor its charge has any overall motion. Since charge must accelerate to emit electromagnetic waves, without such overall motion there can be no emission of electromagnetic waves.
When the 3p electron begins its transition to the empty 3s orbital, however, its quantum wave starts to move. That wave is no longer one motionless standing wave but is, instead, composed of two partial waves: part 3p orbital and part 3s orbital. Because those two partial waves have different energies and different quantum frequencies, they experience time-dependent interference effects. The patterns of constructive and destructive interference change so that the overall electron wave moves rhythmically back and forth and the atom begins to emit an electromagnetic wave. By the time the transition is complete and the electron is entirely in the 3s orbital, the atom has emitted a photon of red light. Like an electron, light travels as a wave but behaves as a particle when you try to locate it. While it's being emitted or absorbed by an atom, light exhibits its particle nature.
We've seen that hot objects emit light, a process called incandescence, but here the neon atom emits light without heat, a process called luminescence. That luminescence is the result of a radiative transition, a transition between quantum states in which a photon of light is emitted or absorbed. In this case, the radiative transition emits a photon that carries away the energy released when the excited neon atom's 3p electron shifts into the empty 3s orbital. The difference in energy between those two states determines the photon energy, which in turn determines the frequency and color of the photon's light wave.
According to quantum physics, a photon's frequency is proportional to its energy. Specifically, the photon's energy is equal to the frequency of its electromagnetic wave times a fundamental constant of nature known as the Planck constant. This relationship between energy is the product of Planck constant and frequency and in everyday language, Ultraviolet light and X-rays can injure your skin and tissues because each particle of high-frequency light carries a great deal of energy.
First used by German physicist Max Planck (1858–1947) in 1900 to explain the light spectrum of a hot object, the Planck constant has a measured value of 6.626 .into. 10 raised to minus –34 Joules ∙ second. Planks constant applies to all quantum waves. For example, the quantum frequency of an electron is related to its energy.
The value of the Planck constant is so tiny that even a photon of 1015-Hz ultraviolet light has an energy of only 6.626 .into. 10 raised to minus –19 Joules. A typical beam of light thus contains so many photons that you can't see that they're arriving as particles. However, the energy in a single ultraviolet photon is substantial on a molecular scale. It can damage a molecule in your skin, contributing to a sunburn and inducing your skin to tan as a defensive response. X-rays have even higher frequencies, and their energetic photons can cause more severe molecular damage.
A neon atom can only emit photons corresponding to energy differences between two of its states, a constraint that severely limits its light spectrum. Neglecting nuclear issues that all neon atoms are identical and emit the same characteristic spectrum of light. The visible part of that spectrum is dominated by the warm red glow of photons emitted when electrons shift from 3p to 3s orbitals, which is why a neon sign glows red.
Since atoms of different elements have different numbers of electrons and different states, each emits its own unique spectrum of light after being excited. Copper atoms emit a blue-green spectrum, strontium atoms a deep red, and sodium a bright yellow-orange. Chemists, astronomers, and manufacturers rely on those emission spectra for information and applications. As we will soon see, so do scientists and engineers working on illumination.
Fluorescent Lamps
If neon tubes appeal to you for illumination, you probably march to your own drummer. Most people opt for a somewhat better simulation of sunlight in their discharge lamps. As an energy-efficient source of artificial sunlight, though, it's hard to beat fluorescent lamps. At the heart of a fluorescent lamp is a narrow glass tube filled with argon, neon, and or krypton gases at about 0.3% of atmospheric density and pressure. The tube also contains a few drops of liquid mercury metal, some of which evaporates to form mercury vapor. About 1 in every 1000 gas atoms inside the tube is a mercury atom, and it's these mercury atoms that are responsible for the light.
Like a neon sign tube, a fluorescent lamp uses a discharge in its gas to produce light. Although fluorescent lamps occasionally use high voltages to initiate their discharges, most operate their discharges at household voltages and must rely on alternative techniques to render their gases conducting. These low-voltage lamps normally heat their electrodes so that thermal energy ejects electrons from their surfaces into the gas. Regardless of how the discharge is started, however, the result is current flowing through the gas and the emission of light.
The fluorescent lamp has a problem, though. While its mercury atoms emit most of the light in its discharge, that light is almost entirely ultraviolet. The final radiative transition that returns each mercury atom to its ground state (6p .to. 6s) releases a large amount of energy and produces a photon with a wavelength of 254 nanometer. This light can't go through the glass walls of the tube, and you couldn't see it if it did. So the fluorescent lamp converts it into visible light with the help of phosphor powder on the inside of the glass tube.
Phosphors are solids that luminesce-they emit light-when something transfers energy to them. Their behavior is similar to that of an atom; an energy transfer shifts the phosphor from its ground state to an excited state, and it then undergoes a series of transitions that return it to its ground state. Some of those transitions are radiative ones and emit light.
In a fluorescent lamp, the phosphor is excited by ultraviolet light. This excitation or energy transfer is actually a radiative transition, but one in which the photon is absorbed by the phosphor; one of its electrons makes a transition from a lower-energy level to a higherenergy level. During this absorption, the light's electric field pushes the electron's wave back and forth rhythmically until it shifts to the new level. The photon disappears, and the phosphor receives its energy.
Once the phosphor is in an excited state, its electrons begin to make transitions back to their ground-state levels. Most of those transitions radiate visible light, the light that you see when you look at the lamp. However, some of the transitions radiate invisible infrared light or cause useless vibrations in the phosphor itself. Despite this wasted energy, phosphors are relatively efficient at turning ultraviolet light into visible light, a process called fluorescence.
The phosphors in a fluorescent lamp are carefully selected and blended to fluoresce over a broad range of visible wavelengths. That blending is necessary because, like atoms, each phosphor fluoresces with a characteristic spectrum of light that's determined by the energy differences between its levels. Several different phosphors are needed to produce the rainbow spectrum of white light. While some advertising and novelty lamps use brightly colored, unblended phosphors, fluorescent lighting tubes use phosphor mixtures that imitate white thermal radiation sources such as the sun or incandescent lightbulbs.
Because compact fluorescent lamps typically produce 60 to 100 lumens per watt, about 4 to 6 times the luminous efficiency of incandescent lightbulbs, you can save a great deal of energy by replacing incandescent lightbulbs with compact fluorescents. In fact, incandescent lightbulbs are being phased out because of their poor energy inefficiency. To ease the acceptance of compact fluorescent lamps by a reluctant public, scientists and engineers have had to improve the quality of their light.
Early fluorescent lamps used a phosphor blend known as “daylight,” which emitted far too much blue light and made everything look cold and medicinal. Daylight lamps rarely found their way into homes. The first improvement came with the developments of the “cool white” and “warm white” phosphor blends. Cool white resembled sunlight, and warm white resembled light from an incandescent lightbulb. However, even these improved lamps didn't emit true white-light spectra-the blackbody spectra of the hot sun or the hot filament of an incandescent lightbulb. Objects looked a little off-color when illuminated by these lamps, so people were hesitant to use them in place of incandescent lightbulbs. Photographers and cinematographers wanting to capture true colors were particularly troubled by fluorescent lighting of that era.
However, the latest generation of fluorescent lamps uses phosphor blends that produce nearly perfect blackbody spectra. Many of those blends are even sold according to their color temperatures. For example, a 5100 K compact fluorescent lamp emits light that is almost indistinguishable from the thermal radiation emitted by a black object heated to 5100 K. That 5100 K color temperature is approximately the color temperature of sunlight averaged over a day. (Sunlight's color temperature is affected by the atmosphere and is highest at noon and lowest at dawn and dusk.) If you like the color of sunlight, use 5100 K compact fluorescents.
People who prefer the warm look of incandescent lighting should choose compact fluorescent lamps with a 2700 K color temperature. These lamps imitate the blackbody spectrum of a 2700 K tungsten filament almost perfectly. Because this 2700 K blackbody spectrum is richer in reds and oranges, it has a comforting, warm character to it. If you can't make up your mind between 5100 K sunlight and 2700 K incandescent light, you can choose many color temperatures in between. Even photographers and cinematographers are now using fluorescent lights, to the delight of actors and news anchors who used to sweat under the intense heat of powerful, energy-inefficient incandescent lamps.
Practical Issues
A fluorescent tube needs a substantial electric field inside it to keep its electrons moving forward. Since this electric field is proportional to the voltage drop through the tube, longer tubes need higher voltages. Power-line voltages (110 to 240 Volts) are appropriate for tubes up to 3 meter in length, but the longer and frequently colored fluorescent tubes used in artwork or advertising require much higher voltages.
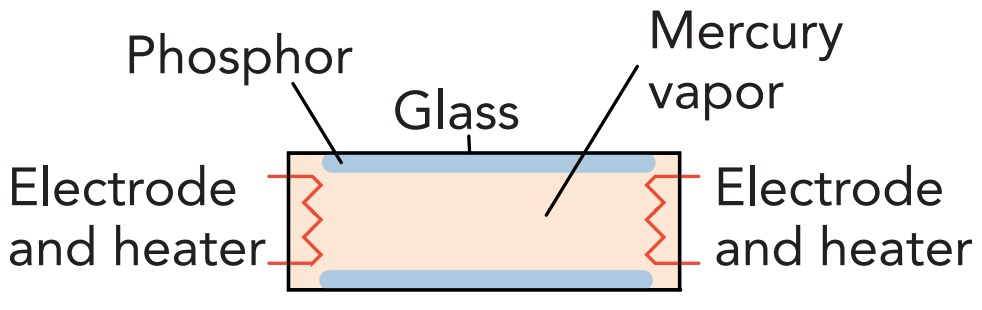
To keep electrons from pushing one another into its walls, a fluorescent tube must also contain positively charged mercury ions. The discharge naturally produces these ions during particularly energetic collisions. The result, a gaslike mixture of positively charged ions and negatively charged electrons, is called a plasma. A plasma is distinct from a gas because its charged particles exert forces on one another over considerable distances. All operating discharge lamps contain plasmas, including the neon sign that we discussed earlier.
Since a typical fluorescent lamp must heat its electrodes red-hot to form its plasma, it runs current through filaments at each end of its tubes. Once the discharge is operating, impacts by electrons may keep the filaments hot enough to sustain the plasma and allow the heating current to be switched off. However, some fluorescent fixtures can be dimmed, in which case electron heating alone won't sustain the plasma. Dimmable fluorescent fixtures must continue to pass heating currents through their filament .or. electrodes.
Each filament .or. electrode is coated with a material that helps it emit electrons into the gas. Unfortunately, that coating is thin and easily damaged by a process called sputtering, in which positive mercury ions from the plasma collide with the coating and chip away its atoms. Once enough of the coating has been removed, the lamp won't be able to sustain a discharge and it will need to be replaced. Because sputtering is particularly severe during start-up, most fluorescent lamps fail after 10,000 to 40,000 starts. Since it takes energy to construct each fluorescent lamp, turning a lamp off for only a short period, a few minutes or less, won't actually save energy in the long run. When you'll be returning to the room in a couple of minutes, leave the fluorescent lights on. Otherwise, turn them off.
Fluorescent lamps operate most efficiently and are at their brightest when their internal temperatures reach about 40 °C. Below that temperature, the vapor pressure of mercury is too low and too much of the lamp's tiny store of mercury is liquid rather than gas. That's why most fluorescent lamps are somewhat dim when you first turn them on and they brighten as they warm up from room temperature to about 40 °C. It takes time for the mercury vapor to reach its optimum density. Although this warm-up period is objectionable to some people, it's a small price to pay for a vastly more energy-efficient lighting.
It would be great if fluorescent lamps could be based on a substance less toxic than mercury. However, mercury is uniquely well-suited to those lamps: its vapor pressure near room temperature is nearly ideal for an electric discharge, it converts electric energy into ultraviolet light with great efficiency, and it isn't damaged or consumed as the lamp operates. Much more important than eliminating mercury from fluorescent lamps is recycling those lamps after they fail. It's not hard to extract and reuse the mercury, and it should be done routinely.
Mercury, Metal-Halide, and Sodium Lamps
Whereas a low-pressure mercury discharge emits mostly ultraviolet light, a high-pressure mercury discharge emits more visible light than ultraviolet light. This change occurs because ultraviolet light becomes trapped in densely packed mercury atoms and only visible light is able to escape from the discharge.
Known as radiation trapping, this effect occurs because mercury atoms absorb 254-nanometer photons just as well as they emit them. The same radiative transition that causes a mercury atom to emit a 254-nanometer photon (6p .to. 6s) can also run backward to absorb that photon (6s .to. 6p). In a dense gas of mercury, whenever one mercury atom emits a 254-nanometer photon, another mercury atom snaps that photon up. So while the discharge keeps pouring energy into the mercury atoms, they can't get rid of it as 254-nanometer photons. Instead, they emit most of their energy through radiative transitions between other excited states. Since this light is much less likely to be captured by other mercury atoms, it emerges from the lamp as bluish visible light.
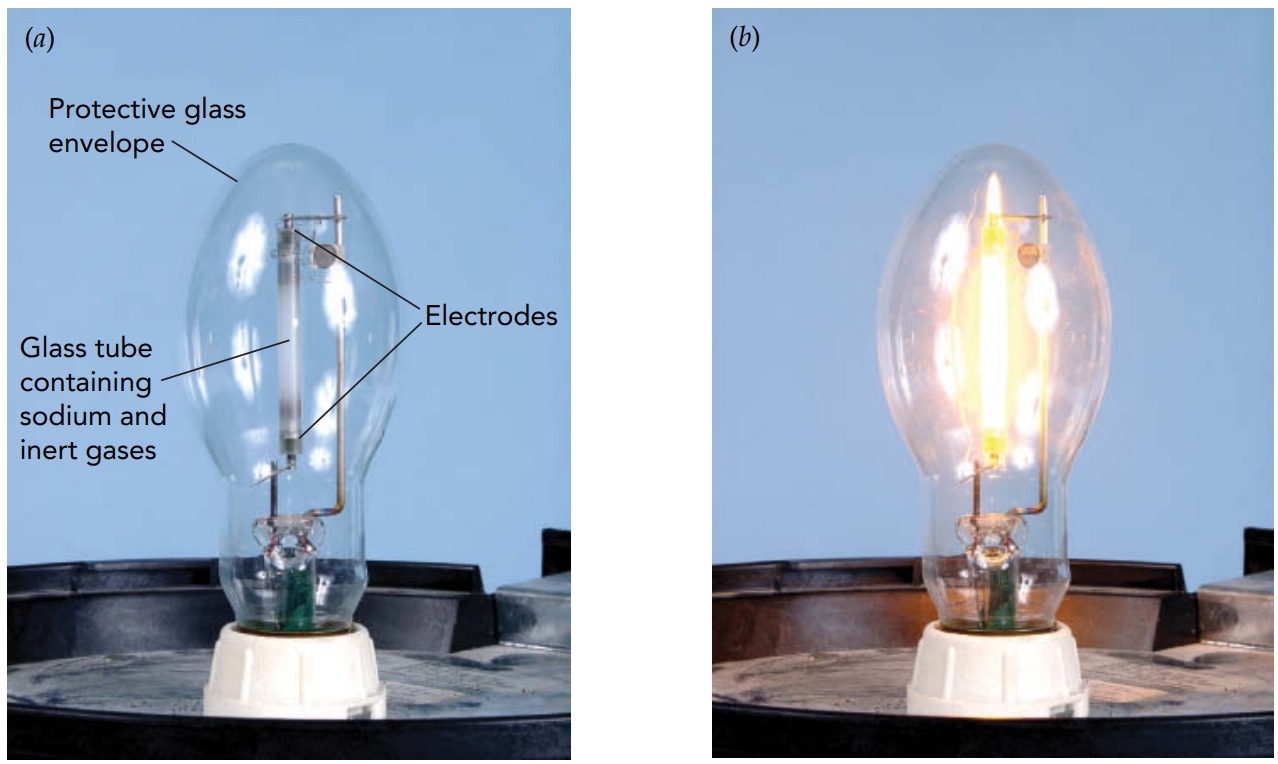
When you first turn on a high-pressure mercury lamp, most of the mercury is liquid and the pressure is low. The lamp starts like a small fluorescent tube without phosphor, so you see very little visible light. However, the tube is designed to heat up during operation so that the liquid mercury evaporates to form a dense gas. As the gas pressure rises, the tube's color changes. The 254-nanometer photons become trapped and photons emitted by many other radiative transitions begin to dominate. The lamp emits brilliant, blue-white light.
To make a lamp that's a little less bluish, some high-pressure mercury lamps contain additional metal atoms. These atoms are introduced into the lamps as metal-iodide compounds, so they are often called metal-halide lamps. Sodium, thallium, indium, and scandium iodides all contribute their own emission spectra to the outgoing light and help to strengthen the red end of its spectrum. They give metal-halide lamps a warmer color than pure mercury lamps. Both high-pressure mercury and metal-halide lamps produce about 50 to 60 lumens per watt, making them almost as energy efficient as fluorescent lamps.
Pure sodium lamps resemble mercury lamps, except that they use sodium atoms. Sodium is a solid at room temperature, so both low- and high-pressure sodium lamps must heat up before they begin to operate properly. A low-pressure sodium lamp is extremely energy efficient because its 590-nanometer light comes directly from a sodium atom's strongest radiative transition. That transition is from sodium's lowest excited state to its ground state (3p .to. 3s). Low-pressure sodium lamps produce almost 200 lumens per watt, which is why highways were frequently illuminated by their yellow-orange glow.
However, this monochromatic illumination is unpleasant and permits no color vision at all. Although it's marginally acceptable on a highway, you wouldn't want it near your home. That's why people buy high-pressure sodium lamps for home use. For a small decrease in energy efficiency, to about 150 lumens per watt, you get a big improvement in color.
Remarkably enough, the 590-nanometer emission itself smears out at high pressure to cover a wide range of wavelengths, from yellow-green to orange-red. This spreading occurs because of the many collisions suffered by the densely packed sodium atoms as they try to emit 590-nanometer light. These collisions distort the atomic orbitals so that the photons emerge with somewhat shifted energies. Overall, a high-pressure sodium lamp emits remarkably little light exactly at 590 nanometer because the ground-state sodium atoms trap that light; they run the (3p .to. 3s) transition backward and absorb the photons (3s .to. 3p). This trapping is so effective that there is actually a hole in the lamp's spectrum right at 590 nanometer.
High-pressure discharge lamps suffer from a problem not found in low-pressure lamps: they're difficult to start when hot. It's much harder to initiate a discharge in a high-pressure gas than in a low-pressure gas, so they all start at low pressure and then evolve to high pressure. If the discharge in a high-pressure mercury, sodium vapor, or metal-halide lamp is interrupted and loses its plasma, the lamp must cool down before it can be restarted.