Outdoor Lights
Today's electronic devices have so many LEDs (light-emitting diodes) that our homes are ablaze with tiny colored lights at night. LEDs are quickly replacing lightbulbs in flashlights, and LED illumination is on the rise. LEDs combine the light-producing quantum transitions of discharge lamps with the solid-state physics of modern electronics. We'll see how electrons behave in solids and how those solids can be tailored to emit light.
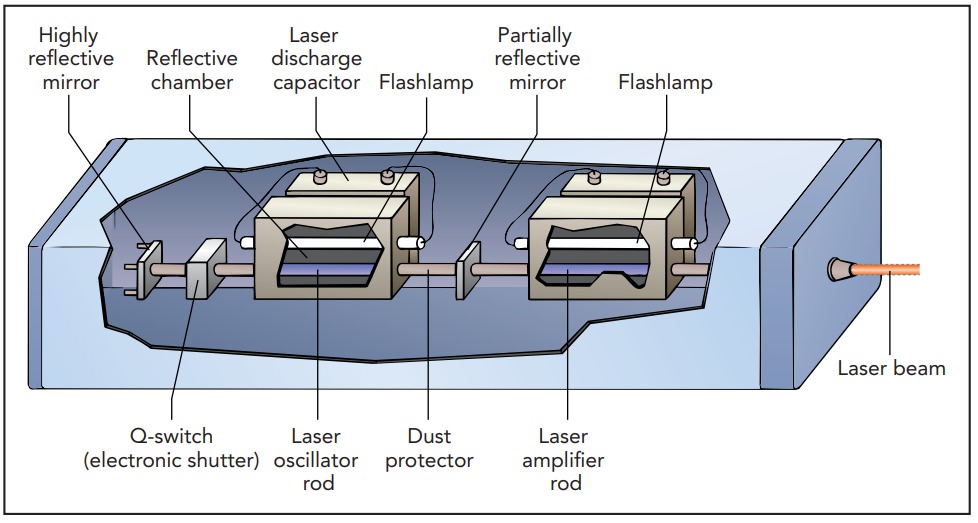
LED
An LED is a solid-state device-that is, it's a solid object that luminesces. Like light from a neon sign, light from an LED is produced when an electron shifts from a higher-energy quantum state to a lower-energy quantum state by way of a radiative transition. In the neon sign, those quantum states involve orbitals, the electron standing waves of an atom. In an LED, however, those quantum states involve levels, the electron standing waves of a solid. To understand how an LED emits light, we must start by understanding levels.
Solids are limited objects, and the basic quantum waves for electrons in limited objects are always standing waves. The electron standing waves in atoms are called orbitals because the positively charge nucleus wraps the negatively charged waves around itself in a manner suggesting orbits. The electron standing waves in solids are less focused on specific atomic nuclei and are called levels instead. Although the nuclei are present, of course, each electron is attracted to all the nuclei at once and that changes the nature of the standing waves.
As we did with orbitals, we'll view levels as abstract placeholders, independent of the electrons that may or may not exhibit them at a given moment. The levels in a solid are then analogous to seats in a theater, each of which may or may not be occupied right now. Instead of saying that the electron is experiencing a particular standing wave or level, we say that the level is occupied by an electron. In this reversed perspective, the level plays the more important role.
Like an electron in an orbital, an electron in a level has a specific total energy-the sum of its kinetic and potential energies. That energy, which depends on the shape and structure of the electron wave, also determines the wave's quantum frequency.
A solid contains an enormous number of electrons, and there are always plenty of levels around to accommodate those electrons. But which levels do they occupy?
At sufficiently low temperature, electrons occupy those levels that have the least energy. For thermodynamic reasons, the electrons settle into the lowest-energy levels available, two electrons per level (the Pauli exclusion principle). By the time all the electrons have been accommodated, they fill the levels up to a certain maximum energy. Halfway between the highest filled level and lowest unfilled level is the Fermi level, a hypothetical level that defines the top of this Fermi sea of electrons. The energy an electron would have in that hypothetical level is called the Fermi energy.
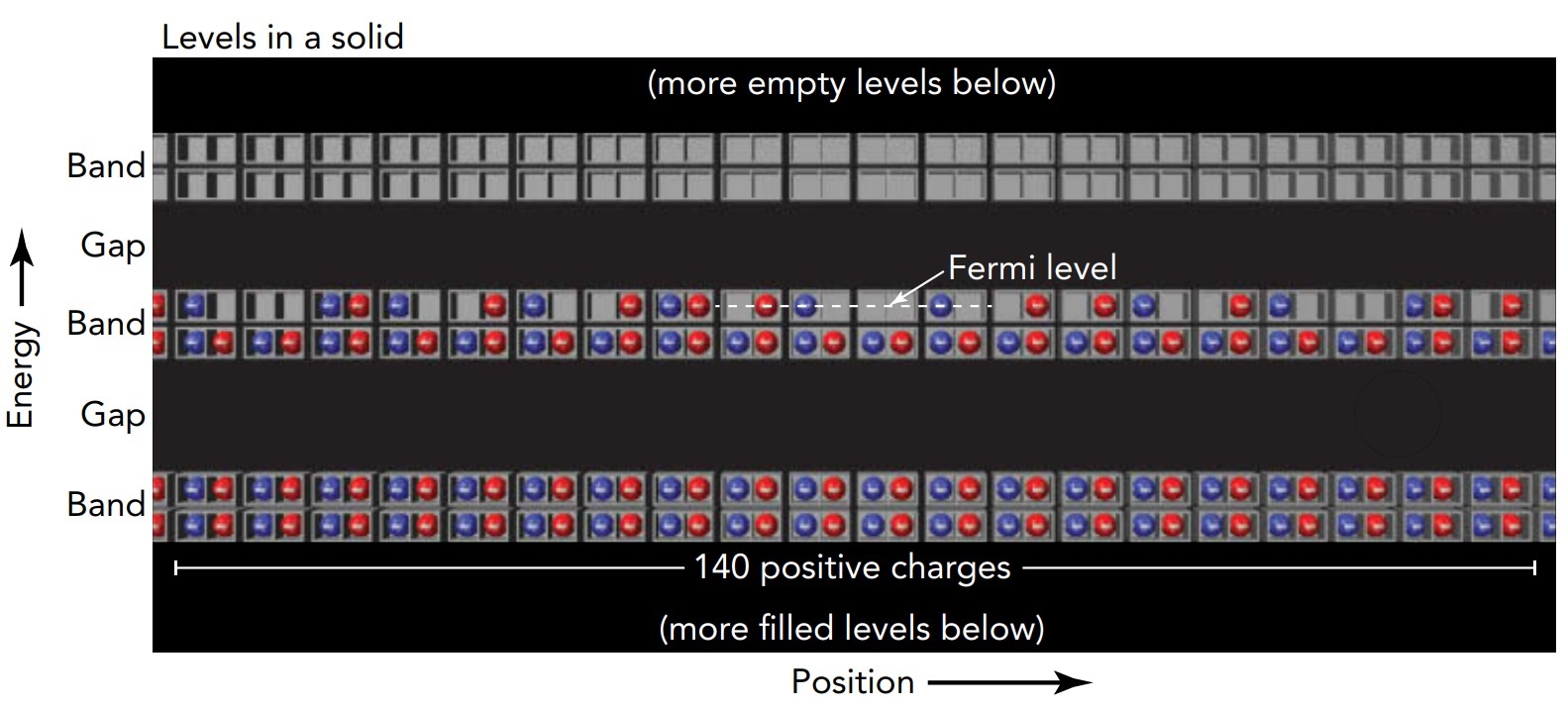
Our theater analogy can again provide insight into this level-filling process. It is analogous to what happens at a popular show: the seats fill from the orchestra level on up- everyone wants to sit in the lowest (and closest) seat. When the show starts, people have filled all the seats up to a certain highest seat. Halfway between that last filled seat and the next unfilled seat is the hypothetical Fermi seat.
If we represent the levels graphically by boxes and arrange them vertically according to energy, then the levels below the Fermi level contain two electrons each, while those above the Fermi level are empty. Although thermal energy complicates this picture somewhat by shifting electrons about near the Fermi level, we can ignore that detail near room temperature or below.
Since levels are standing waves, they don't have sharply defined locations in space. However, we can safely imagine that each level places its electrons near a particular location in the solid. Although this picture is somewhat oversimplified, it's accurate enough to illustrate much of the physics of charge motion in materials.
Of course, electrons aren't the only charged particles in a solid. The atoms also have positively charged nuclei. But those nuclei are essentially immobile and rarely participate in the flow of electricity.
Metals, Insulators, and Semiconductors
The levels in a solid occur in groups called bands. Each band corresponds to standing waves with a particular type of structure. Since the levels in a band involve similar waves, they also involve similar energies. Between these bands of levels there are sometimes band gaps, ranges of energy in which no levels exist. The solid does not and cannot contain electrons with energies that lie within a band gap. Bands and band gaps are what distinguish metals, insulators, and semiconductors. When the Fermi level is located in a band gap, it can prevent the electrons in a solid from responding to outside forces. To see how that happens, let's examine first a metal and then an insulator.
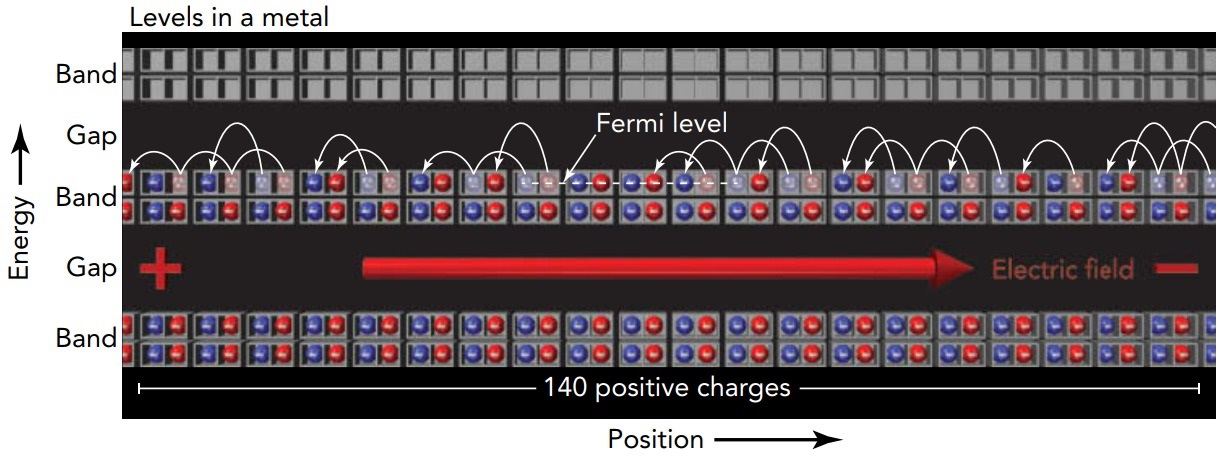
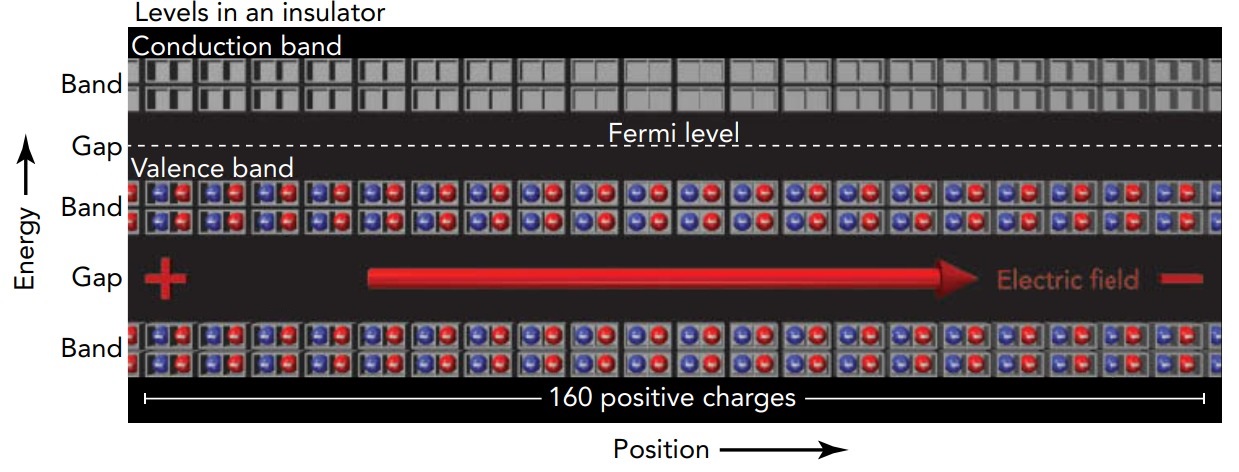
In a metal, the Fermi level lies in the middle of a band. Because the band's empty levels are just above its filled levels, very little energy is needed to shift electrons from the filled levels to empty levels. This feature allows the metal to conduct electricity. When you subject the metal to a voltage difference, giving its left side a higher voltage than its right side, the resulting electric field points toward the right. That field pushes the metal's negatively charged electrons toward the left and they being to move left. They move by shifting from filled levels to empty levels, obtaining the energy needed to reach those empty levels from the work done on them by the electrostatic forces. Overall, electrons enter the metal from its right and leave from its left, so the metal conducts electricity
In our theater analogy, a metal is a theater in which only about half the ground-floor seats are filled. If you ask people in the theater to begin shifting left, those near the top of the occupied seats can shift about easily. Each finds an empty seat nearby on the left and moves over. New people are then able to enter the theater from the right while others leave the theater from the left. This metal theater is conducting people. Unlike the situation in a metal, in an insulator the Fermi level lies in the middle of a band gap, between the top of one band and the bottom of another band. With no easily accessible empty levels available, a great deal of energy is required to shift electrons from the filled levels to empty levels. When you subject the insulator to a voltage difference, higher voltage on the left, the resulting electric field points toward the right and pushes the insulator's electrons toward the left. Those electrons, however, have no empty levels nearby and are thus unable to move. To shift into one of the empty levels in the upper band, an electron in the lower band would need more energy than it can get from the electrostatic forces.
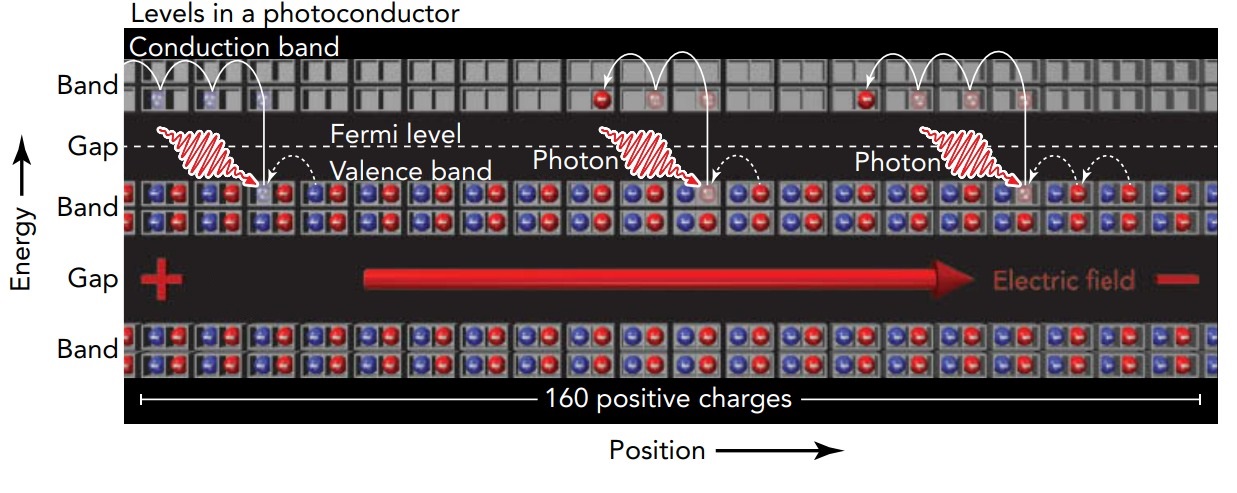
Since no net charge flows through the insulator, it doesn't conduct electricity! In our theater analogy, an insulator is a theater in which all the ground-floor seats are full and the balcony seats are empty. When you ask people in this theater to begin shifting left, they can't do it. All the ground-floor seats to the left are filled, and people can't reach the balcony to make use of its empty seats. This insulator theater is unable to conduct people. In a metal, the band of levels containing the Fermi level is only partially filled, and electrons can easily shift from filled to unfilled levels. In an insulator, the band below the Fermi level-the valence band-is full and the band above the Fermi level-the conduction band-is empty, making such shifts extremely difficult. Even in an insulator, however, an electron can shift from a valence level to a conduction level ((a level in the valence band to a level in the conduction band) if something provides the necessary energy. One such energy source is light. When an insulator is exposed to the right type of light, that light can shift electrons from the material's valence band to its conduction band.
Once electrons appear in the normally empty conduction band and empty levels appear in the normally full valence band, electrons can respond to electric fields. They can shift from filled levels to nearby empty levels and thus travel through the material. Electrons can then enter the material from one side and leave from the other, so the material conducts electricity. Because light has made this insulator a conductor, we call the material a photoconductor.
Not all light causes photoconductivity in an insulator. Light is emitted and absorbed as particles called photons. Each photon's energy is proportional to its frequency; the higher the frequency of light, the more energy each of its photons contains. To shift an electron across the large band gap in a typical insulator, high-energy, high-frequency light is needed; the insulator must be exposed to violet or even ultraviolet light.
Nature also provides materials with smaller band gaps that can be crossed with the help of low-energy, low-frequency red or even infrared light. These materials are called semiconductors because their properties lie somewhere between those of conductors and insulators. Semiconductors have small band gaps, making it relatively easy for light, heat, or other types of energy to shift electrons between valence and conduction levels. In our analogy, a semiconductor theater is an insulator theater with a low balcony, so that even a baby gorilla can toss people into it.
For more than half a century, scientists and engineers have worked with semiconductors to produce an astonishing array of electronic devices. By carefully tailoring the shapes and chemical compositions of semiconducting materials such as silicon, germanium, and gallium arsenide, they have created virtuoso instruments for electron waves in solids that are every bit as remarkable as the instruments for musical waves found in great orchestras. Of all these electronic instruments, the simplest is the semiconductor diode.
Diodes
A diode is a one-way device for current; it allows current to flow through it in one direction but not in the other. Although diodes have taken many forms over the years, the diodes in virtually all modern electronic devices are built from semiconductors. A basic semiconductor diode is made by joining together two different semiconductors. These two semiconductors have been modified so that they don't have perfectly filled valence levels and perfectly empty conduction levels. Instead, they're doped with atomic impurities that either create a few empty valence levels, p-type semiconductor or place a few electrons in the conduction levels, n-type semiconductor. These empty valence levels or conduction-level electrons allow p-type and n-type semiconductors to conduct electricity.
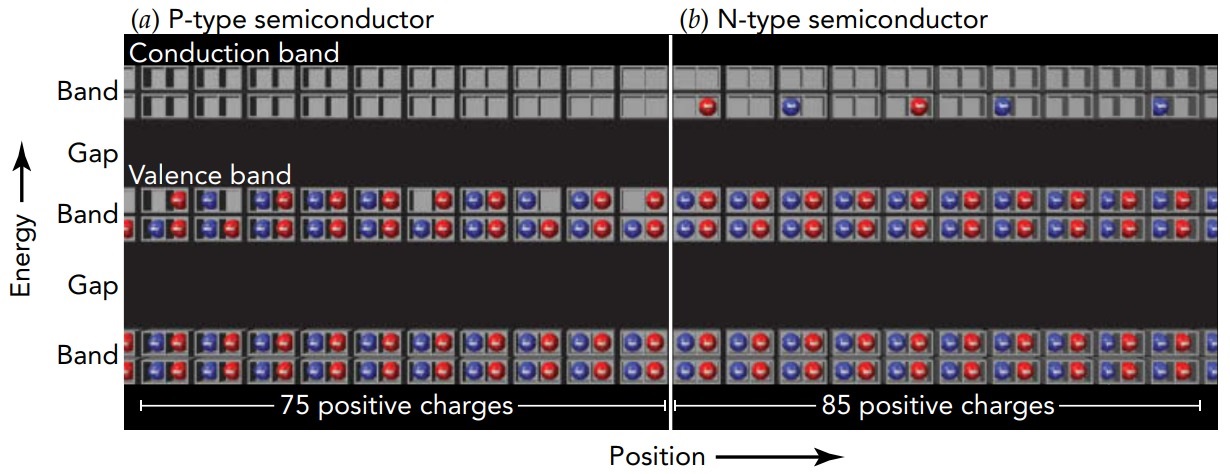
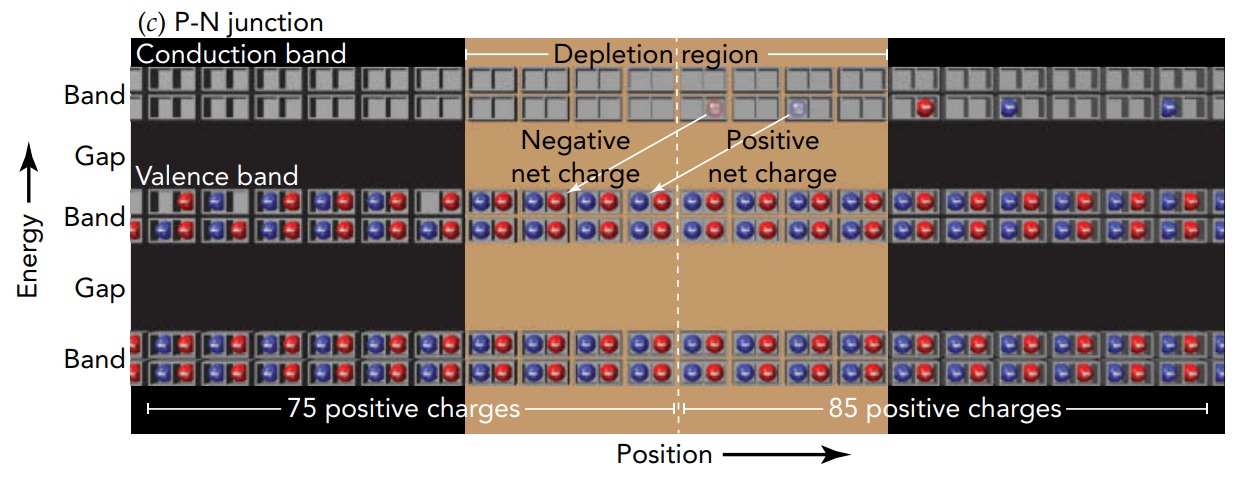
When a piece of p-type semiconductor touches a piece of n-type semiconductor, however, something remarkable happens-a p-n junction forms at the place where the two meet. To reduce their potential energies, higher-energy conduction-level electrons from the n-type semiconductor migrate across the p-n junction to fill empty lowerenergy valence levels in the p-type semiconductor. It's an example of static electricity; when two dissimilar materials touch, the material that is hungrier for electrons (the p-type semiconductor) steals some electrons from its partner (the n-type semiconductor).
As the electron migration proceeds, the n-type semiconductor acquires a positive net charge because it now has fewer electrons than positive charges. The p-type semiconductor acquires a negative net charge because it now has more electrons than positive charges. Electrostatic forces from this separated charge oppose the further migration of electrons across the junction and gradually bring that migration to a halt. Everything is then in equilibrium.
Near the p-n junction, there is now a depletion region, an area in which electron migration has emptied all the conduction levels and filled all the valence levels. With no conduction-level electrons or empty valence levels left, the depletion region can't conduct electricity and charge can't move across the p-n junction. The depletion region is an insulator, and the two pieces of semiconductor have become a diode. In our theater analogy, the p-n junction is analogous to a theater with two halves. In the left or “p-type” half, the balcony is empty and even the ground floor has some empty seats. In the right or “n-type” half, the ground floor is filled and there are even a few people in the balcony. Since these two halves touch, people in the right balcony notice the empty seats in the left ground floor, and a few of them near the center of the theater clamber down from the right balcony to the left ground floor to take advantage of the better seats. Near the center of the theater, the ground floor is now filled and the balcony is empty, forming a depletion region in which no one can move left or right. The central part of the theater can't conduct people!
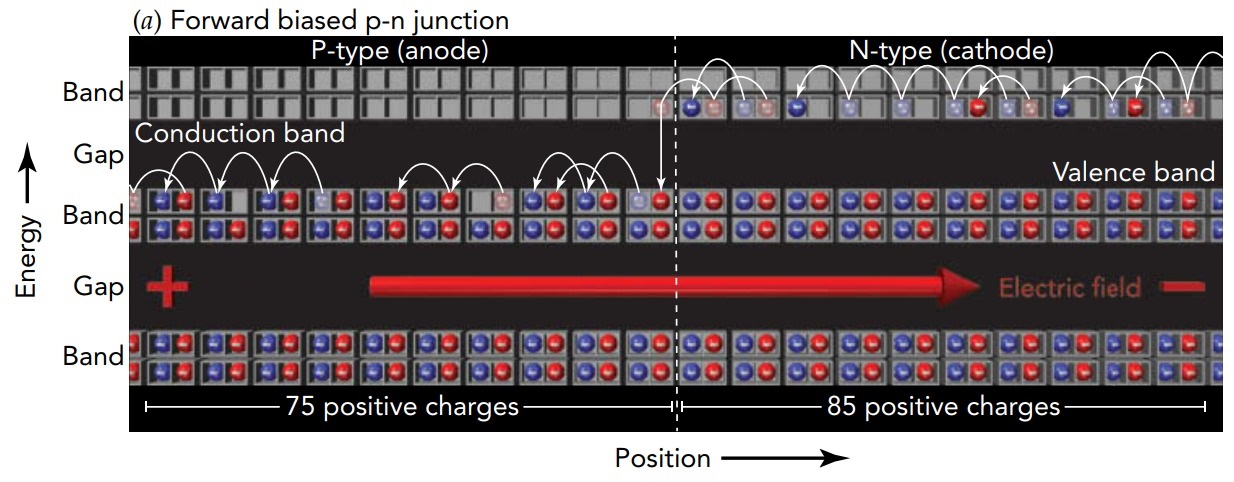
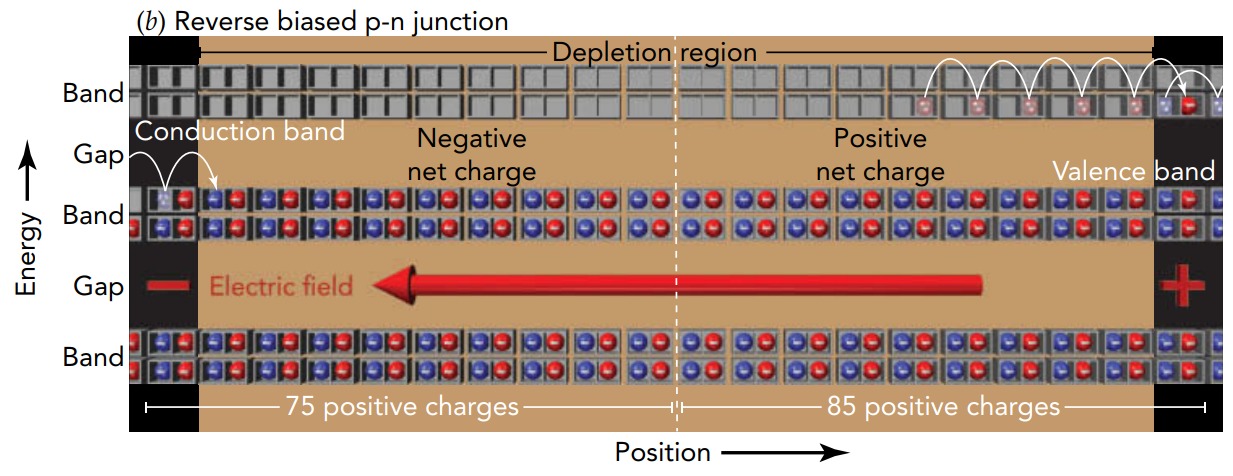
Let's now look at what happens when we attach wires to each semiconductor half and use a battery to impose a voltage difference between those two halves. The voltage of the p-type side is higher than the voltage of the n-type side, so the resulting electric field points toward the right and pushes electrons toward the left. Electrons migrate toward the p-n junction in the n-type side's conduction levels and away from the p-n junction in the p-type side's valence levels. At the same time, some electrons enter the n-type side from its wire and some electrons exit the p-type side to its wire. The depletion region becomes thinner and, when the voltage difference is large enough, vanishes altogether. Once that happens, electrons begin to cross the p-n junction and the entire device and it conducts electric current.
In the theater analogy, we're adding people on the right to the n-type balcony and removing them on the left from the p-type ground floor. The new people in the n-type balcony can move about the empty seats and migrate toward the center of the theater. Similarly, the empty seats in the p-type ground floor allow people to shift about so that empty seats become available near the center of the theater. At that point, people in the n-type balcony can cross over to the p-type balcony and then jump down to the ground floor. There is a net leftward flow of people through the theater; the theater is conducting people from right to left.
What happens if we reverse the battery? The voltage of the n-type side is higher than the voltage of the p-type side, so the resulting electric field points toward the left and pushes electrons toward the right. In this case, the depletion region becomes thicker as the electrons fill in the empty valence levels in the p-type side and leave the conduction levels in the n-type side. The widening depletion region prevents charge from moving and no current flows across the p-n junction. The device remains an insulator. In the theater analogy, we're removing people on the right from the n-type balcony and adding them on the left to the p-type ground floor. Soon the n-type balcony is virtually empty and the p-type ground floor is essentially full. The entire theater is now a depletion region and behaves like the insulator theater. No one can move and the theater can't conduct people.
Since it allows current to flow in one direction but not the other, the p-n junction is a diode. For historical reasons, the diode's p-type side is called the anode and its n-type side is called the cathode. Current, which is the flow of positive charge, can pass through a diode only from its anode to its cathode. Since current naturally flows from higher voltage to lower voltage, a diode carries current only when it is forward biased, that is, when its anode has a higher voltage than its cathode. When it is reverse biased, when its anode has a lower voltage than its cathode, no current flows through the diode.
Light-Emitting Diodes
Even when a diode is forward biased, its depletion region doesn't vanish until the voltage of its anode is significantly higher than the voltage of its cathode. For the ordinary silicon diodes commonly used in AC adapters and computer power supplies, a voltage drops of 0.6 Voltsis required before they'll conduct current. Since the current passing through the diode is then experiencing a voltage drop, the diode is consuming electric power. Because the diodes used in adapters and power supplies serve only as switches to control the direction of current flow, the electric power consumed by these diodes is simply converted into thermal power. But some specialized diodes are designed to convert much of the electric power they consume into optical power-they emit light!
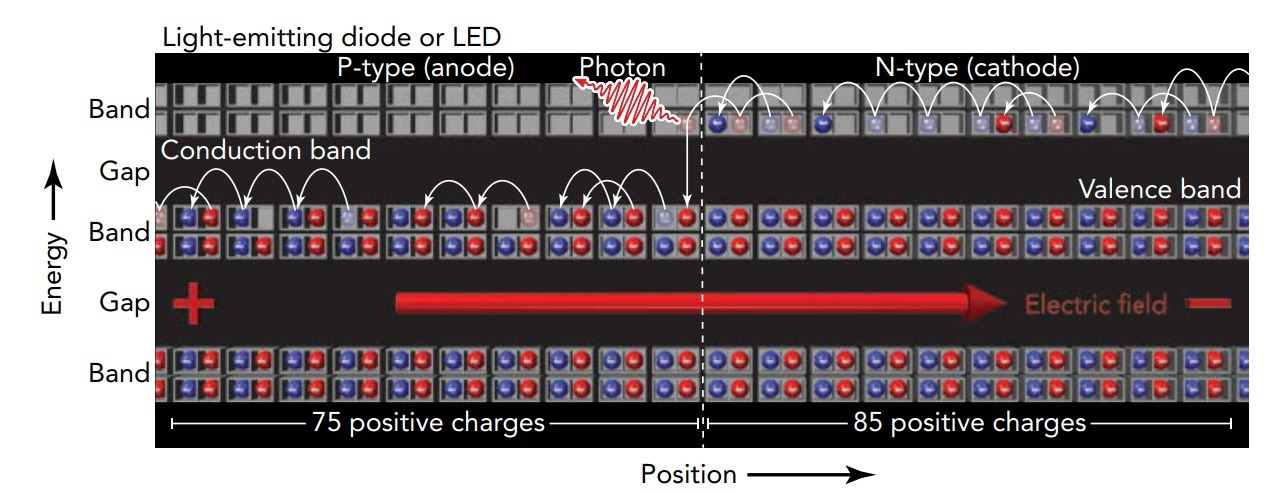
When a diode is forward biased and current flows from its anode to its cathode, conduction-level electrons in the n-type cathode travel across the p-n junction to become conduction-level electrons in the p-type anode. In effect, the anode is then in an excited state; it has conduction-level electrons and empty valence levels. What happens next depends on the characteristics of the diode. In a normal silicon diode, those conduction-level electrons shift to empty valence levels without producing significant light. Silicon's band structure has characteristics that discourage light emission, so most of these electron transitions produce internal vibrations and heat the diode instead of producing light.
In specialized diodes made from more exotic semiconductors, conduction-level electrons injected from the n-type cathode into the p-type anode frequently undergo radiative transitions to empty valence levels and thereby emit light. Composed primarily from combinations of gallium, indium, aluminum, arsenic, phosphorus, and nitrogen, they are known as light-emitting diodes, or LEDs. LEDs now come in just about any color of the rainbow, including infrared, red, orange, yellow, green, blue, violet, and ultraviolet. Although white LEDs are also common, they're actually violet or ultraviolet LEDs with built-in phosphors that fluoresce white.
An LED's color is directly related to the energy released when an electron in its p-type anode shifts from a conduction level to a valence level. The most convenient unit in which to measure that energy is the electron volt (abbreviated e V), the energy released when 1 elementary unit of electric charge experiences a 1-Voltsdecrease in voltage (1 electron Volts is equal to 1.6021 .into. 10 raised to minus –19 Joules). In a typical red LED, an electron releases 1.9 electron Volts as it shifts from a conduction level to a valence level and can produce a photon with an energy of 1.9 electron Volts. Also, 1.9-electron Volts photon has a frequency of 4.6 into 10 raised to 14 Hz and a wavelength of 650 nanometer.
To operate and produce these 1.9-electron Volts photons, the red LED must be forward biased with a voltage drop of at least 1.9 Volts. The current-carrying diode uses that voltage drop to inject electrons into the anode's conduction band, where they have energies 1.9 electron Volts above the valence band. Many of those electrons subsequently release their excess energies as 1.9-electron Volts photons of light.
The shorter the wavelength of the light an LED emits, the more energy each electron must release as it shifts from a conduction level to a valence level and the larger the semiconductor's band gap must be. A violet LED that emits 400-nanometer light requires a band gap of about 3.1 electron Volts to produce its 3.1-electron Volts photons. That LED also needs a forward-bias voltage in excess of 3.1 Volts. The larger voltage drops required by LEDs near the violet end of the spectrum explain why those LEDs need higher-voltage power sources or more batteries.
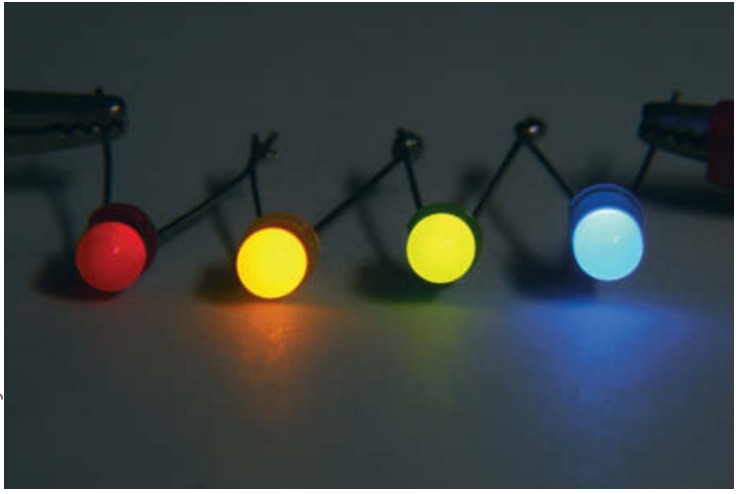
Unfortunately, at most about half of the electrons sent across an LED's p-n junction succeed in lighting the room. Although a substantial fraction of those electrons emit photons, most of the photons are reabsorbed by the semiconductor before they leave the LED; the same radiative transitions that emit this light (conduction level .to. valence level) can also absorb it (valence level .to. conduction level). Despite those difficulties, modern LEDs can produce visible light with energy efficiencies comparable to those of fluorescent lamps. LED efficiencies continue to rise along with their operating lifetimes, and they are gradually becoming a primary form of illumination.